Engineering Biology Aspirations
Updated 25 April 2025
Engineering Biology Aspirations Foresight report April 2025
This is not a statement of government policy.
Preface
This report is about the power of engineering biology to drive economic growth and deliver enduring solutions to a wide range of complex problems in areas such as healthcare, environmental sustainability, agriculture and energy.
Put simply, engineering biology is the application of engineering principles to biological systems in ways that allow us to harness and control biology in predictable and useful ways. For example, to create novel products and processes that are safer, cleaner and more efficient.
As with other emerging technologies, communicating the benefits of engineering biology can be challenging due to the complex terminology involved. Our experience from previous Foresight projects is that a good way to cut through this barrier to greater understanding is to focus on the practical benefits and solutions offered by a technology via narratives that capture the imagination.
I have therefore commissioned a set of 5 evidence-based case studies covering a diverse range of sectors and applications. The papers are intentionally aspirational in terms of their outlook for the future of engineering biology and focus on providing an optimistic, yet realistic, vision of what engineering biology can do to help solve some of our enduring challenges. The aim is that these examples, together with their supporting chapters, will act as a source of inspiration across government and the public, for what might be possible if we can harness the opportunities offered by engineering biology.
I hope that this report will demonstrate the breadth of engineering biology’s transformative potential to solve real-world problems and help to communicate the benefits of engineering biology beyond communities already familiar with the technology. Finally, I want to thank the experts who wrote the papers and who contributed more broadly to this report.
Professor Dame Angela McLean Government Chief Scientific Adviser
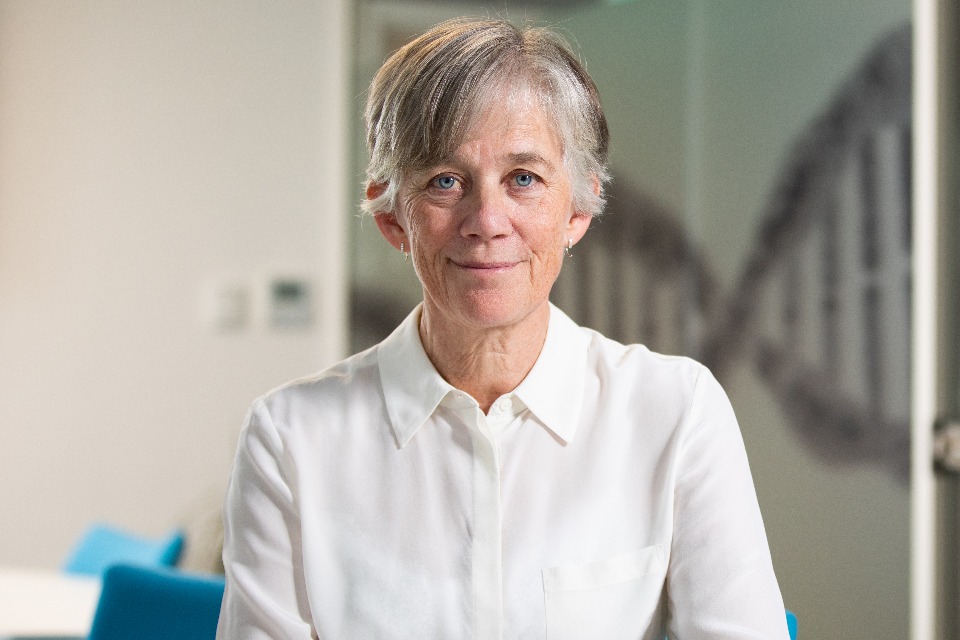
Image of Professor Dame Angela McLean, Government Chief Scientific Adviser, sitting at a desk.
Executive summary
Engineering biology (EngBio) is a fast-moving field of science with the capability to provide solutions to many of society’s enduring problems.
This report highlights the potential applications of EngBio across a range of settings via a set of expert-authored ‘aspiration papers’. These papers are intended to give the reader an optimistic overview of what the future of EngBio might look like across 5 distinct areas, and how it could help to transform multiple industry sectors over the coming years.
An AI generated, futuristic agricultural facility with cylindrical buildings covered in greenery and wind turbines. The landscape includes fields of crops, solar panels, and scientists in lab coats. Drones fly above, indicating automated systems. The scene suggests eco-friendly and innovative agriculture.
Figure 1: Engineering Biology offers a world of possibilities (AI-generated image).
Background
EngBio is a form of biotechnology that combines principles from biology, engineering and computer science to design, construct and commercialise new or modified biology-derived products and services. The goal is to create organisms or biological systems with novel functions that can address challenges in areas such as medicine, agriculture, and environmental sustainability.
EngBio is closely related to synthetic biology, the field of science in which biological components such as organisms and bio-molecules are redesigned to have new characteristics, using tools such as gene editing. EngBio takes the products of synthetic biology and applies principles drawn from the world of engineering, such as the ‘design, build, test, learn’ (DBTL) cycle, to create alternative and novel products and processes across a range of industrial sectors.
However, EngBio is not just about the application of synthetic biology — it also draws on techniques from other disciplines, such as metabolic engineering, to modify existing organisms. Likewise, while genetic modification is a major tool in engineering biology, not all EngBio involves genetic modification. The field also includes non-genetic techniques, such as bioprocess engineering, to manipulate and optimise biological systems via processes such as fermentation, without altering the genetic makeup of the organisms involved.
EngBio has the potential to manufacture new products in ways that are kinder to the environment and that offer greater efficiencies when compared to more traditional methods. Examples include alternatives for fossil fuels, industrial dyes and clothes that are less harmful to the environment, more effective medicines, greener ways of feeding ourselves, and methods to convert or recycle waste into useful products.
In short, EngBio is the science of manipulating biology to create solutions to the problems that people and the planet face, now and tomorrow.
Approach
The aim of this report is to communicate the potential benefits that EngBio might bring to our society and economy over the next 10 years and beyond. To do this, we commissioned leading experts to write 5 case studies exploring what we consider to be among the most exciting and potentially transformative applications of EngBio. These case studies have been deliberately selected to cover a diverse range of sectors and applications, and demonstrate the breadth of EngBio’s transformative potential to solve real-world problems.
Each paper begins with an aspirational statement describing the goals of EngBio and envisioning a successful world in 2035. The papers then outline the problem at hand and how EngBio provides a solution. They offer a brief overview of the necessary steps to achieve the goal, addressing any potential challenges or societal considerations that need to be overcome. Finally, they conclude with a statement on the long-term impact of the EngBio solution.
The papers are summarised below and can be found in full from Chapter 3. To supplement the aspiration papers, we also spoke to a selection of Chief Scientific Advisers (CSAs), including the Government Chief Scientific Adviser (GCSA), to seek their views on the future of EngBio. The outcomes of these discussions are reflected in Chapter 3 and a more detailed record of the discussions can be found in Appendix A.
Aspiration papers - summaries
Bio-Synthetic Fuels: Imagine a world where clean, fossil-free fuels can be produced right where they are needed. Redesigning microbes to manufacture sustainable fuels and chemicals from waste.
Nitrogen-fixing Cereals: Imagine a world where humanity’s main source of carbohydrates – cereal crops like wheat and barley – are able to generate their own nitrogen fertiliser.
Engineering a new generation of crops to tackle global food shortages and drive forward sustainable agriculture.
Future Fashion: Imagine a world where every piece of your clothing comes at minimal cost to the environment.
Harnessing the power of microorganisms to create cleaner and safer ways of making our clothes, footwear and accessories.
Lab-Grown Blood: Imagine a world where no patient dies due to a lack of compatible blood during surgery, accidents, or medical crises.
Creating a safe and unlimited source of blood for all.
Microbial Metal Factories: Imagine a world where we’ve solved metal scarcity.
Forging a sustainable future for the metals our industries rely on.
Conclusions
This report does not make specific policy recommendations, but it does set out a vision for what the future of EngBio might look like along with some of the challenges for the future, in a format we hope readers will find helpful. The intention is to inspire senior officials and policymakers and help them to understand and communicate the future potential of EngBio. Key points are as follows:
-
The future of EngBio is incredibly promising, with the potential to revolutionise various sectors, from healthcare to environmental sustainability.
-
By addressing key challenges such as public acceptance, regulatory frameworks, and skills development, we can harness the power of biology to create innovative solutions that benefit society and the environment. The UK’s commitment to this field, through strategic funding and international collaboration, positions us to lead in this transformative area.
-
As we move forward, it is essential to maintain strong leadership, foster interdisciplinary collaboration, and ensure robust public engagement. By doing so, we can build a thriving EngBio industry that not only drives economic growth but also contributes to a healthier, more sustainable world.
Finally, we should embrace what EngBio has to offer and work together to unlock the potential of EngBio for the betterment of all.
How to use this report
The primary audience for this report is senior officials and policymakers across government departments who may be aware of the term engineering biology, but not how it might be relevant to their particular area of work. For these readers, the report provides some tangible examples of how EngBio might be applied across a wide range of settings.
By publishing the report on gov.uk we also want it to be read by the wider public with the intention that it should help communicate some of the ways in which EngBio could solve societal problems over the next 10 to 15 years.
We hope you find this report useful. It’s important for us to monitor the impact our work has so we’d appreciate it if you’d contact us at foresight@go-science.gov.uk to let us know how this report has informed and influenced your work.
1. Introduction
Defining Engineering Biology
Engineering biology (EngBio) applies engineering principles to the design of biological systems and processes. This interdisciplinary field integrates techniques from biosciences, engineering, and physical sciences, with synthetic biology at its core. Synthetic biology involves creating biological components, systems, and materials from biological elements for innovative purposes. EngBio uses engineering techniques to convert these concepts into practical solutions for societal challenges, addressing issues faced by people and the planet, now and in the future.
A circular diagram divided into 4 quadrants: Design, Build, Test, and Learn. Each quadrant contains icons and text describing activities such as DNA libraries, gene cloning, microfluidics, and AI. The diagram illustrates the phases of a process from design to learning.
Figure 2: Examples of key capabilities underpinning engineering biology (Council for Science and Technology, 2023)
The engineering principle at the heart of EngBio is the ‘design, build, test, learn’ (DBTL) cycle that has long been a central element of product development in more traditional engineering disciplines. The complex and often unpredictable nature of biological systems creates the need to test multiple permutations to obtain the desired outcome, requiring an iterative process to drive innovation.
DBTL in EngBio means designing and building a biological system, be it some sort of genetically-engineered system, or cell, or biological process; testing it to see how well it works; and then feeding in the learnings from this analysis to another round of design, iteratively improving the system. The cycle incorporates many different capabilities and disciplines including gene editing, modelling and AI and machine learning – see Figure 2 for examples of key capabilities underpinning EngBio and the DBTL cycle. Crucially, this extends beyond gene editing. In fact, many examples of exciting and innovative research and development in this area contain no genetic manipulation at all.
Historical context
Although ‘engineering biology’ is a relatively new term, it is by no means a new discipline. We have been modifying biology for about 12,000 years with farming, which has sought to continuously improve on the biology of crops and domesticated animals through selective breeding, thus harnessing it for our advantage. In the mid-19th century, with scientific breakthroughs such as Darwin’s Theory of Evolution and Mendel’s cross-pollination experiments, we started to understand the mechanisms by which beneficial characteristics are passed from generation to generation.
Fast forward to the middle of the 20th century, where our understanding extended down to the molecular level. Starting with Watson and Crick’s discovery of the structure of DNA, we began to be able to manipulate and transfer genetic material from one organism to another to create the first genetically modified organisms. In the 21st century, we now have the ability to redesign biological components for novel purposes in the multidisciplinary field of synthetic biology. The industrial application of synthetic biology, in combination with rational engineering principles, to create new products brings us to the present day and the emergence of what we know as engineering biology.
A brief history of Engineering Biology’ highlights significant milestones from 1859 to 2020. Key events include Darwin’s theory of evolution (1859), Mendel’s genetics research (1866), the discovery of DNA’s structure (1953), the creation of the first genetically engineered organism (1973), the commercial availability of human insulin (1982), the invention of PCR (1985), the mapping of the human genome (2000), the creation of the first synthetic cell (2010), the development of CRISPR-Cas9 (2012), and the release of AlphaFold (2020).
Figure 3: A timeline depicting some of the key milestones across the history of engineering biology to date.
EngBio opportunities
EngBio has the potential to provide solutions to some of society’s biggest and most longstanding challenges, spanning through healthcare and personalised medicine, environmental sustainability, sustainable agriculture, and energy security. These solutions include the creation of alternative and novel products, and processes that have the potential to be more efficient and more environmentally sustainable. They include sustainable aviation fuels, industrial dyes and clothes with massively reduced carbon footprints, more effective medicines, and safer, greener consumer products. Through these advances, EngBio promises economic growth, job creation and improved quality of life.
EngBio also has significant economic potential that could help drive economic growth in the UK. In their 2020 report ‘The Bio Revolution’, the McKinsey Global Institute estimated that between 2030-2040, as scientific, regulatory and commercial challenges are overcome, EngBio could account for up to $2.2 trillion per year in terms of direct annual impact. The report also estimates that as much as 60% of the world’s physical inputs (such as materials and fuels) could be produced using biological means (McKinsey & Company, 2020).
McKinsey further noted in their 2021 report on the UK biotech sector (of which EngBio is an integral part) that the UK has founded more biotechnology companies than any other nation in Europe (McKinsey & Company, 2021). DSIT analysis from 2023 estimates there to be around 1000 EngBio firms in the UK (Department for Science Innovation and Technology 2023b).
Advances in various foundational and interdisciplinary technologies, including DNA sequencing, proteomics, gene editing tools, bioinformatics, and AI, have significantly driven the progress in engineering biology and are expected to become increasingly important. In particular, the convergence of AI and engineering biology offers great potential for future increases in predictive designing and optimisation of biological systems. The UK already has world-leading capabilities in this space, as demonstrated by the 2024 Nobel Prize in Chemistry that was shared by some of the UK’s brightest minds behind Google DeepMind’s protein structure prediction tool, AlphaFold. Continuing to pursue developments in these technologies will help to broaden the range of biological products at our disposal, as well as the rate at which we are able to produce them, fostering increased growth and innovation across the UK.
The UK’s strengths
Engineering biology, and before it, synthetic biology, have been an area of focus for the UK government with an emphasis on early investment and a proactive approach to responsible and trustworthy innovation. In 2012, the Synthetic Biology for Growth programme committed £70 million, rising to £102 million, on a programme of investment including 6 research centres around the UK (UK Research and Innovation, 2023). Since then, EngBio has been named as one of five critical technologies for delivering prosperity and security to the UK in 2023’s Science and Technology Framework (Department for Science, Innovation and Technology, 2023a) due to existing capabilities within the UK and its potential to revolutionise the bioeconomy. The National Vision for Engineering Biology was subsequently published in 2023, reiterating government’s ambition for the sector and providing a framework for the future direction of government investment, policy and regulation (Department for Science, Innovation and Technology, 2023b).
The UK’s strong research base in the life sciences and capabilities underpinning EngBio, such as AI, together with industrial innovation, government support, and a collaborative approach to solving global challenges helps to ensure the UK is among the world leaders in EngBio. Recent Government Office for Science (GOS) analysis for the period 2019 to 2023 shows that the UK has strengths in research, patents and early commercialisation, but performs less well in scaling up. Further GOS analysis indicates that there is good potential for the UK to develop domestic productive capacity in EngBio, given the specialised capabilities we hold and the economic potential of the sector, and that this is more promising for EngBio than for other emerging technologies. However, with other countries investing heavily and starting to reap the benefits of EngBio, the UK’s advanced position within the field is not guaranteed over the long term (House of Lords, Science and Technology Committee, 2025).
A year of EngBio
Over the last 12 months, the Government Chief Scientific Adviser (GCSA), Dame Angela McLean, has made it her mission to expand the evidence base around the applications and benefits of EngBio and to proactively communicate these insights across government and the public, as part of her ‘Year of Engineering Biology’.
This Foresight report is by definition deliberately forward-looking and focuses on the future of this field. By engaging widely with experts across the field, we were able to reveal a huge variety of exciting opportunities and applications that this technology could offer in the next 10 years and beyond. 5 of the most exciting and potentially transformative areas of research are explored in more detail in this report from the perspective of leading experts working in the field. These aspirational visions for the future of EngBio have been deliberately selected to cover a diverse range of sectors and applications, and they demonstrate the breadth of EngBio’s transformative potential to solve real-world problems.
These ‘aspiration papers’ are intended to provide tangible examples of the future potential of EngBio in a way that will resonate with those who have no specialist knowledge of EngBio. They are not statements of government policy but rather are intended to inspire readers and showcase the scale of what might be possible if we can harness the opportunities offered by EngBio. For this reason, they are optimistic in terms of their future outlook for EngBio.
This approach is in no way meant to diminish the challenges faced by EngBio, such as those around scale-up, public perception, skills and investment. These are already covered in detail by other reports, such as the National Vision for Engineering Biology (Department for Science, Innovation and Technology, 2023b), and indeed, the aspiration papers each include a section on hurdles and societal considerations. Instead, we want this report to set an inspiring, yet realistic vision of what EngBio can do to help solve some of our enduring challenges. The focus is therefore on how EngBio can open the door to solving future challenges, potential impacts, and how EngBio can transform national capability.
We hope this report will serve as a valuable resource for policymakers, guiding their decisions on the future of EngBio. Through this project and the Year of EngBio, we aim to foster new workstreams, stimulate discussions, and increase references to EngBio. Our goal is to enhance understanding of the terminology and generate excitement and momentum around EngBio.
2. Engineering biology aspirations
The case studies presented in this report are central to showcasing the transformative potential of EngBio. They illustrate 5 distinct visions, spanning various sectors and applications. These examples highlight EngBio’s ability to address real-world challenges effectively. Our goal is to inspire both government and the public by demonstrating the possibilities that can be realized through harnessing EngBio’s opportunities.
Criteria for selecting the papers
In selecting these 5 examples, we wanted to communicate the applications of EngBio, and the solutions it can offer, to senior stakeholders and policymakers across government, as well as the general public. To achieve this, we devised the following criteria for selecting 5 topics that should:
- broadly cover the following sectors: agriculture and food, chemicals and materials, health, defence, and national security and resilience;
- centre on a specific example of a transformative application of EngBio that could come to fruition in 10 to 15 years;
- showcase examples of EngBio providing a solution to a real-world problem, preferably offering a clear advantage over other possible solutions;
- represent a mix of applications from the business and academic communities; and
- be presented in a way that is simple to grasp and can capture the imagination and inspire readers.
Using these criteria, we consulted stakeholders across government and the scientific community to identify the following topics and corresponding authors:
- Bio-Synthetic Fuels – Professor Nigel Scrutton (University of Manchester and C3 Biotech)
- Nitrogen-Fixing Cereals – Professor Philip Poole (University of Oxford)
- Future Fashion - Jen Keane and Dr Ben Reeve (Modern Synthesis)
- Lab-Grown Blood – Professor Ash Toye (University of Bristol and Scarlet Therapeutics)
- Microbial Metal Factories – Professor Louise Horsfall (University of Edinburgh)
Structure of the papers
The intention is that these papers should capture the imagination and communicate the future potential of EngBio. They are therefore future-focused and invite the reader to imagine a world in which EngBio has solved a particular real-world problem.
Each paper starts with a short aspirational statement outlining what researchers are trying to achieve in EngBio, and how the world might look in 2035 if they are successful. The papers then outline the general problem we are trying to solve and how EngBio offers a solution, before providing a brief overview of what needs to be done to achieve the goal, and any challenges or societal considerations that might need to be overcome. Each paper concludes with a statement of the long-term impact of the EngBio solution.
These papers have been authored by EngBio experts selected from the business and academic communities. As such, they represent the opinions of those authors and are not a statement of government policy. Furthermore, this report does not offer any endorsement of the companies mentioned in the papers. Any reference to a specific company is made in the context of explaining a particular EngBio application.
2.1 Bio-Synthetic Fuels
Redesigning microbes to manufacture sustainable fuels and chemicals from waste.
Imagine a world where clean, fossil-free fuels can be produced right where they are needed. Using microorganisms and the power of engineering biology, waste materials can be turned into fuel for homes and businesses, recycling carbon rather than removing it from the ground, creating a more sustainable circular economy. This could have huge benefits for applications in defence, farming and humanitarian missions. Local microbe-powered fuel factories could also be game changing for remote areas like islands where transporting fuel is difficult and costly. Microbe-powered factories can help to meet a diverse range of energy needs and build a more sustainable future.
Current status
Carbon emissions from burning fossil fuels are the main cause of global warming (IPCC, 2022). To reduce these emissions, many sectors are transitioning to electrical power, which can be supplied by renewable sources such as wind and solar. But some applications are much more difficult to electrify — the batteries used in electric vehicles are too heavy, and carry too little energy, to power long-distance air travel, for example (Schäfer, 2018)
Aviation relies almost exclusively on kerosene-based fuels, which account for roughly 3% of global CO2 emissions per year (European Commission, 2024). These emissions could be curbed by sustainable aviation fuels (SAFs), liquids that are similar to today’s jet fuel, but synthesised from agricultural residues or other waste biomass (US Department of Energy, 2023). Yet despite its potential, SAF accounted for only 0.3% (roughly 1 million tonnes) of global aviation fuel consumption in 2024, and production is projected to reach only 17.3 million tonnes annually by 2040 (Higgins, 2024). Current SAF technologies also do not address the logistical challenges and high costs associated with transporting liquid fuels, particularly to remote regions.
Distributing fuels from refineries to end users generates CO2 emissions — even before the fuel is burned — which undermines net-zero ambitions due to the large volumes of materials moved over vast distances (Greene, 2020). Transporting fuel is also expensive: within the US military, for example, the estimated cost of a single gallon of kerosene can soar from $3 at the point of manufacture to between $100 and $600 at the point of use, depending on locations and distribution challenges (Erwin, 2010).
In this context, producing kerosene and related fuels at the same locations they are needed could provide a transformative solution. This kind of distributed production could be achieved by engineered microorganisms, designed to generate fuels by digesting locally-sourced waste feedstocks such as indigenous biomass, agricultural waste, or industrial, food and municipal wastes. Crucially, by recycling carbon from waste, this approach offers a route to a more sustainable circular economy for fuels. This approach would significantly reduce both the logistical and environmental costs of long-distance fuel transport.
Engineering biology opens the door
Microorganisms, such as bacteria, are miniature chemical factories, turning simple molecules such as amino acids or sugars into a wide range of other biochemicals necessary for life. The series of biochemical reactions involved in each transformation are guided and accelerated by biological catalysts called enzymes. The tools of synthetic biology enable us to redesign these microbes to help us meet a diverse range of energy needs.
For example, it is possible to engineer a type of bacteria called Halomonas to turn the amino acids in food waste or brewery residues into a mixture of propane and butane — key components of liquefied petroleum gas (LPG), widely used as a fuel for heating and cooking (Amer 2020a; 2020b). These microbes can also be engineered to make valuable chemicals that can be turned into plastics (Faulkner, 2023). Crucially, Halomonas are a very robust type of bacteria which thrives in salty environments, meaning that the fermentation process to make these useful chemicals can be carried out in non-sterile seawater rather than having to create and maintain the exacting conditions used in typical biomanufacturing plants. This makes it a cheap, convenient and energy-efficient production system.
The UK is already pioneering approaches to apply this exciting fundamental research to real-world challenges. C3 Biotech are a spin-out from the University of Manchester who are harnessing the power of microbes to convert sugary raw materials, such as food waste, into synthetic kerosene for aviation fuel. They have already piloted this approach to successfully power a drone in a 20-minute demonstration flight, in collaboration with the Ministry of Defence (Ministry of Defence, 2022). Given the UK defence interest in hypersonic flight (Wright, 2023; Ministry of Defence, 2024), there is the potential for this approach to be used in future to supply high-performance fuels to meet these needs.
There are clear environmental benefits to creating such synthetic fuels which are entirely fossil fuel-free. However, one of the other advantages is the portability of this technology. The process for producing these fuels does not require large-scale infrastructure, meaning that bioreactor plants could be assembled in shipping containers to create modular fuel production systems. Enabling fuels to be produced locally and on-demand could have numerous benefits: making fuel production more equitable and affordable, reducing the logistics costs of fuels operations, and reducing dependence on volatile international oil markets and supply chains. It could also substantially reduce the carbon emissions that are currently incurred by transporting fuels.
Mobile fuel platforms could also be scaled and configured to suit different local needs by harnessing the power of engineering biology. Microbes could be used to first biosynthesise fuel precursor molecules and then by selecting different chemical processes, these precursors could be converted into a variety of different fuel components. This simple two-stage approach could help to create different fuel formulations with desired performance characteristics. Examples could include converting agricultural or industrial wastes into valuable chemicals or fossil-free fuels such as low-temperature diesels, which could be used for aviation, ground transport and generators. This has the potential to revolutionise energy access in remote regions and transform industries worldwide.
A diagram showing bio-synthetic fuel production. The first is a microorganisms, then a fermentation tank. It then shows a bulk container where the fuel is kept and then multiple contains are shown that can be shipped. Finally, it shows an example of where it can be used, a drone.
Figure 4: A simplified diagram representing the process of producing bio-synthetic fuel. This starts with microorganisms (1), such as bacteria, which when grown in lab fermentation conditions (2) can produce chemicals for sustainable fuels. These reactions can be compartmentalised at increasing scale through intermediate bulk containers (3), large containers (4), up to mass storage and transportation of microbial fuel factories (5). This has the potential to provide sustainable sources of fuel for a diverse range of energy requirements, with aviation fuel being one example (6).
The work ahead
The pilot plant that has been developed by C3 Biotech to produce synthetic kerosene using microbes is the first of its kind and provides a model for developing mobile systems to produce cleaner fuels. Translating this foundational R&D into scalable, commercial energy solutions, is likely to require the integration of engineering biology with techniques from process engineering and process chemistry:
Engineering biology: ‘industrialising’ suitable microorganisms to ensure that they can be controlled in a stable and reliable way to feed on a wide range of available feedstocks and produce suitable amounts of a desired product. Feedstock availability will also need to be carefully considered as this could present a challenge on the route to commercial viability.
Process engineering: improving the compatibility of component units for waste handling and product recovery, as well as designing innovative new biological reactor systems to house the non-sterile seawater fermentation processes.
Process chemistry: early milestones should focus on achieving cost reductions through better catalyst design and other innovations.
Repeated cycles of design, prototyping, demonstration, verification, roll out and replication for scaled production will be key to industrialising this technology. Achieving overall cost reductions and enhanced process stability will be crucial. Artificial intelligence (AI) could play a pivotal role in accelerating progress, particularly in process engineering, by filling data gaps and enabling faster decision-making and system optimisation.
On the journey towards scaling-up bio-synthetic fuel production, a prototype installation could be used to understand overall performance and integration with existing infrastructure in different locations. This prototype could be located at a UK military base or platform, or at a suitable industrial location with access to biomass waste and links to fuel users. An early and important goal would be to produce sufficient fuel (possibly around 500 litres) for testing and certification by an independent body such as the UK SAF Clearing House (UK SAF Clearing House, n.d.).
A subsequent milestone could be to establish a larger mobile fuel system using multiple units at a UK industrial region, aiming to produce roughly 10,000 litres of fuel per week. This would require full integration of all the components needed to convert biomass waste into fuel precursors, and subsequently into bio-synthetic fuels such as kerosene. Spent biomass waste would need to be recycled, for example into biogas, to support the plant’s energy requirements and also generate surplus energy. Techno-economic and life cycle assessments should be carried out throughout all stages of the programme, to assess cost-effectiveness and sustainability.
For these fuel systems to be truly mobile, the components will need to be containerised. Transporting and deploying such a system for performance testing at an overseas UK military base for example, or an equivalent facility, will be a key step in demonstrating the utility of this technology for local fuel production and waste management in more remote overseas locations.
Potential challenges and societal considerations
Industrialising these bio-synthetic fuel factories is likely to require substantial investment to fund engineering works. Companies such as C3 Biotech are aiming to structure implementation of the technology so that investments are able to drive the delivery of key milestones and outcomes as the technology matures. The ambition is that this would also facilitate the attraction of new capital, through mechanisms such as joint ventures, equity shares, or similar arrangements.
As with other emerging applications of engineering biology, public perceptions will need to be carefully considered as the technology matures. There should be a detailed evaluation of the public acceptability of using engineered organisms to produce fuel intermediates at scale, and in different settings. This will help to gauge the appetite for such technologies and identify any areas where increased transparency or education might be beneficial.
Safety and reliability are critical in the fuels sector, and rapidly deploying new technologies in this field presents significant challenges. One major advantage of mobile bio-synthetic fuel factories is that they rely on relatively small, transportable, containerised units that could be quickly deployed and replicated to meet specific application needs. This would not only facilitate early deployment of safe and reliable operational units, but also enable real-world testing of production economics and operation before committing to larger-scale capital investments, thereby mitigating financial risk. This approach would provide early production capabilities in settings such as UK overseas territories or for defence and humanitarian applications, where rapid fuel delivery can be particularly complex and challenging.
A transformative national capability
The development of mobile factories for producing and deploying clean fuels on demand could make significant contributions towards meeting UK Net Zero and energy security targets, facilitating decarbonisation across multiple sectors and industrial and domestic sites in the UK and overseas. One example is enabling the transition to clean fuels at UK industrial sites that handle nuclear waste or lack gas energy infrastructures.
The mobility of these fuel platforms would also be particularly well-suited to locations where there are changing energy needs, or where it’s not possible to quickly develop alternative renewable infrastructure such as wind turbines or solar panels. The rapid deployability of the technology is well suited to support humanitarian initiatives, and it could potentially drive economic growth in remote island nations and overseas territories. These fuels could also play a key role in defence applications, such as large drone operations, stealth aircraft, and hypersonic flight, whilst supporting both national and NATO commitments.
Given the strong foundational research base in the UK, including companies such as C3 Biotech, this technology might be deployable in several years ahead of the longer timelines of up to 15 years that are projected for conventional SAF programmes. Through the use of a circular economy model that uses localised waste materials, the vision set out by C3 Biotech could help to achieve the UK’s commitment of reducing economy-wide greenhouse gas emissions by at least 81% by 2035, compared to 1990 levels (UK Government, 2025). Deploying this technology would tap into locally available bio-waste, unskilled labour, saline or contaminated water, and low-cost engineering materials, while offering significant environmental, health, and economic benefits. Bio-synthetic fuels therefore represent a potentially transformative application for engineering biology which could advance the UK’s global leadership on climate change, while simultaneously creating jobs and helping to grow the economy.
2.2 Nitrogen-Fixing Cereals
Engineering a new generation of crops to tackle global food shortages and drive forward sustainable agriculture.
Imagine a world where humanity’s main source of carbohydrates — cereal crops like wheat and barley — are able to generate their own nitrogen fertiliser. This would solve one of the biggest challenges in agriculture, improve crop yields around the world, and bring enormous environmental benefits. Cereals currently require industrially produced nitrogen fertilisers that are a major contributor to global warming, and which cause catastrophic nitrogen pollution in waterways. Engineering biology offers radically new ways to develop self-fertilising cereals, paving the way to more sustainable agriculture.
Current status
Plants cannot grow without nitrogen — it is an essential element in amino acids, proteins, DNA, and most other biological molecules required for life. Although nitrogen gas makes up nearly 80% of the Earth’s atmosphere, it is chemically inert and cannot be used directly by plants. To unlock this nitrogen for plants, nitrogen gas must be converted into a more chemically active form, such as ammonia. This process is called nitrogen fixation.
Soil bacteria known as rhizobia are particularly adept at nitrogen fixation, because they contain an enzyme called nitrogenase that can break the strong chemical bonds in nitrogen gas to make ammonia (Poole, 2018). To exploit this source of biologically available nitrogen, legumes such as peas, beans, clover and soybean attract rhizobia to take up residence in the plants’ specialised root nodules. In return for a steady supply of ammonia, the plants house and feed the bacteria, forming a remarkable symbiotic relationship. Legume seeds can be coated with rhizobia to increase yields, and the bacteria may also be added directly to fields, a process called soil inoculation.
However, cereal crops like wheat and barley do not benefit from such fruitful partnerships. These plants do not possess root nodules, nor can they produce the special signalling chemicals that legumes use to encourage rhizobia. The classic strategy to overcome this deficiency is crop rotation: farmers plant cereals in fields where legumes once grew, so that the cereals can absorb residual ammonia and other active forms of nitrogen that the legumes left behind in the soil. Crop rotation also improves soil health, by building up soil carbon and reducing microbial pathogens. However, the use of legumes in crop rotation has suffered a serious decline in many Western countries, perhaps due to the relentless pressure to maximise crop yields.
Instead, the most common solution is to spread ammonia-based chemical fertilisers onto fields, so that cereal crops can absorb all the nitrogen they need directly from the soil. Organic materials such as manures can also be added to farmland for their fertilising properties, and to ensure healthy soils which are resilient to environmental change. However, it is the artificially produced chemical fertilisers that have enabled substantial increases in food production, albeit with significant environmental impacts.
Globally, farmers use more than 200 million tonnes of chemical fertilisers every year (FAO, 2019). The ammonia in these fertilisers is made from nitrogen gas using the industrial Haber-Bosch process, which consumes about 1% to 2% of the world’s energy supply, and causes a similar proportion of global CO2 emissions (Kyriakou, 2019). Once fertiliser has been applied to fields, it can release the potent greenhouse gas nitrous oxide. Rain can also wash fertiliser into waterways, where it feeds blooms of algae and plants in a process called eutrophication. These proliferating organisms suck up oxygen from the water, killing fish and other species (Steffen, 2015).
To avoid the expense and environmental damage of chemical fertilisers, agricultural researchers have spent decades trying to endow cereals with the same nitrogen-fixing capability that benefits legumes.
The concept sounds simple, yet the biology and technology involved is complex. While recent scientific advances are promising, nitrogen fertiliser-free cereal crops are still a distant prospect. Various research programmes are already working to speed up this eminently desirable development (Geddes, 2015; Haskett, 2020; Jhu, 2023; Mus, 2016). But engineering biology now offers a step change in our ability to develop nitrogen-fixing cereals.
Engineering biology opens the door
The anatomy and biochemistry of legumes are fundamentally different to those of cereals. The relationship between legume and rhizobia is also finely regulated by complex genetic networks in both the plant and the bacteria, which orchestrate the exchange of multiple chemical signals to control the symbiotic interaction. These factors make it challenging to use conventional genetic modifications to imbue cereals with the ability to fix nitrogen.
Research on this problem therefore requires a multidisciplinary approach, involving genetics, biochemistry, microbiology and soil ecology. Engineering biology is vital to this effort, because it enables various genetic and biochemical processes in both plants and bacteria to be isolated and then reassembled in novel ways, so that they can be introduced into new organisms such as cereals. The biochemical networks in these modified cereals can then be tested to study their effects, enabling further improvements. This work hinges on the key engineering biology principle of using ‘design, build, test and learn’ cycles.
Researchers are currently taking several different approaches to achieve nitrogen fixation by cereal crops. The first strategy is to create cereals that can fix nitrogen directly from the air, so that they no longer need symbiotic bacteria. This involves taking key genes from rhizobia that are involved in nitrogen fixation, and adding them to the leaves or roots of the crop. These genes contain instructions for the biochemical machinery that performs nitrogen fixation, and they also help to regulate that machinery while it operates. Achieving this feat would be a revolutionary breakthrough in plant biology, but the complicated molecular biology and biochemistry involved really makes this a long-term strategy (Burén, 2017; Lopez-Torrejon, 2016).
The second approach is to engineer cereals to form nodules on their roots that can host nitrogen-fixing bacteria. The UK is the global leader of this strategy, thanks to support from UK Research and Innovation. The University of Oxford is part of this effort, funded through an Engineering and Physical Sciences Research Council (EPSRC) Engineering Biology initiative. There is also a major program on developing nodulating cereals led by the University of Cambridge, backed by a large investment from the Gates Foundation and the UK’s Foreign, Commonwealth and Development Office.
These teams all work together as part of a large collaboration, and have recently made some significant advances. For example, they have engineered several non-legume plants, including the cereal barley, to form nodule-like structures. Meanwhile, barley roots have also been engineered to release a chemical signal called rhizopine (Geddes, 2019) that prompts rhizobia to start fixing nitrogen (Haskett, 2022; Haskett, 2023).
In these experiments, the bacteria reside on the surface of the barley’s roots. The long-term aim is to generate nodules on cereal roots — including barley, wheat and maize — that are able to host rhizobia. This would create a ‘synthetic symbiosis’ that offers a powerful way to increase nitrogen uptake by the plants.
Diagram showing 3 routes for nitrogen fixation in cereal crops. The first has a plant with engineered nodules and one without. The next has a plan with engineered signalling molecules on the root and one without. And lastly, there is a plan with nitrogen-fixing bacteria within the soil and one without.
Figure 5: 3 possible routes that are being explored to achieve nitrogen fixation in cereal crops. (A) Engineering cereals to form nodule-like structures on their roots that can host nitrogen-fixing bacteria. (B) Engineering cereals to release chemical signalling molecules from their roots which can attract nitrogen-fixing bacteria. (C) Engineering nitrogen-fixing bacteria to persuade them to enter cereal root nodules. Some combination of all 3 approaches may be the most likely route to success.
The work ahead
To create this kind of synthetic symbiosis, researchers need to persuade rhizobia to enter the cereal’s root nodules. That means both plant and rhizobia will have to be engineered to control the rhizobial infection process.
Engineering biology is extremely well suited to tackling this challenge, because it can employ multiple rounds of modifying, testing, and redesigning the organisms involved in order to coordinate the complex plant-microbe signalling mechanisms. Although this represents a long-term goal, coordinating bacterial activity and nodule formation would be a game-changer for nitrogen-fixing cereals and global agriculture.
Rhizobia are not the only nitrogen-fixing bacteria in soil: species of Azotobacter, Klebsiella, Gluconacetobacter and Kosakonia can also convert atmospheric nitrogen into ammonia (Dixon, 2004). These bacteria often live in soil immediately adjacent to plant roots, a region known as the rhizosphere. There, the roots provide the bacteria with carbohydrates for energy, while the bacteria return the favour by supplying biologically-available nitrogen to the plant.
Cereals could be genetically engineered to interact more efficiently with these bacteria in the rhizosphere. This approach would be easier to achieve than synthetic symbiosis, but may be limited by competition from other resident soil bacteria.
Given all of these efforts, it is not unrealistic to expect substantial progress in the coming years, and proof-of-principal engineered cereals could be developed by 2035. However, it could still be several decades before cereals can be engineered to fix more than 50% to 75% of their total nitrogen requirement.
Potential challenges and societal considerations
The complexity of the genetic engineering in this area means that success cannot be guaranteed. It is imperative to understand that the transformative possibilities of engineering biology are real, but that the timelines required to make progress are likely to be medium- to long-term. These difficulties mean that there is still uncertainty over the most suitable approach to pursue. For now, it would be sensible to continue a combination of the various programmes of research.
That is not to say that engineering biology cannot also provide near-term solutions to other challenges in agriculture. For example, the Genetic Technology (Precision Breeding) Act, which passed into law in March 2023 in the UK, allows farmers to use gene editing to grow crops which are more resilient to drought and disease, helping to increasing food security (Department for Environment, Food & Rural Affairs, 2023).
As nitrogen fixation research continues, it will be important to consider any societal concerns around the production and release of genetically-engineered plants and microbes as well as backlash from those that may interpret such approaches as ‘tampering with nature’. Some concerns could be alleviated through increasing awareness and understanding of the science underpinning this research. For example, the fact that engineered rhizobia would only be able to fix nitrogen when working in partnership with the engineered, nodulated cereal, and not with other plants, could help to mitigate cross-contamination concerns.
Nevertheless, this is a complex challenge. In order to harness the full potential of this technology to solve food security and sustainability challenges, it will be vital to bring the public along the entire journey from research through to deployment.
A transformative national capability
The development of these self-fertilising crops would be a step change for developing countries, where even small amounts of nitrogen fixation by cereals could have significant positive impacts on grain yields. In turn, this would result in more nutritious diets, income generation, and poverty alleviation.
In developed countries, access to cereals with high rates of nitrogen fixation could significantly reduce fertiliser use, resulting in a substantial decrease in agricultural greenhouse gas emission and nitrogen pollution of ground water. Reducing nitrogen run-off would reduce eutrophication of waterways and coastal areas, preventing the formation of oxygen ‘dead zones’.
Engineering biology offers the opportunity to tackle one of agriculture’s biggest and most longstanding challenges. By creating cereal crops that do not rely on chemically produced nitrogen, there is the opportunity to leave behind industrially-produced and environmentally-polluting nitrogen fertiliser whilst simultaneously tackling global food shortages.
2.3 Future fashion
Harnessing the power of microorganisms to create cleaner and safer ways of making our clothes, footwear and accessories.
Imagine a world where every piece of your clothing comes at minimal cost to the environment. Your sweater is made of fibres and yarns produced from regenerative resources, and your shoes are assembled locally with components from a resilient supply chain. The adhesives, foams, and trims used in your bag are bio-based and recyclable in a single process, with zero waste going to landfills. Even if a piece of clothing is accidentally discarded into the environment, it safely biodegrades to leave no trace of its existence. This is the future of fashion, and engineering biology is helping to make it happen.
Current status
The fashion industry is one of the world’s largest polluters. It is responsible for 2% to 8% of the world’s climate-warming carbon emissions (UNEP, 2022), and 20% of global clean water pollution is caused by dyeing and treating textiles (European Parliament, 2024). Over half of fashion’s environmental footprint comes from its raw materials (Fashion for Good, 2020), so the industry is unlikely to meet its climate pledges (Mehta, 2023) without sourcing more sustainable alternatives.
The vast majority of clothing is made from synthetic materials derived from fossil fuels. These materials are not biodegradable and can pose significant environmental and health risks. Polyester and polyamide, for example, which constitute over 60% of global fibre production (Textile Exchange, 2024), shed plastic microfibres during washing, daily wear, and recycling. These microfibres pollute water sources, harm ecosystems, and have even been found in human bloodstreams, where they may have adverse health effects (Leonard, 2024). Discarded garments often end up in landfills where they can persist for decades or centuries, potentially leaching harmful chemicals and greenhouse gases as they degrade (Cuiffo, 2021).
Animal-derived leathers are another important category of primary material for the fashion industry. Yet leathers raise ethical concerns regarding animal welfare, and involve resource-intensive production processes with a considerable environmental footprint. Unfortunately, plastic-based alternatives often lack the durability of natural leather.
Today, as few as 3% of clothes worn in the UK are manufactured domestically (Partington, 2022), and the UK textile industry has suffered a significant decline due to underinvestment and offshoring. This lack of investment has made it difficult for domestic manufacturers to compete with their foreign counterparts, in terms of efficiency and cost.
Recognising these challenges, pioneering brands in the fashion industry are beginning to explore new materials. These range from plant-based alternatives, such as mushroom leather and pineapple leaf fibres, to recycled materials and innovative textile blends. However, many of these solutions offer only incremental improvements or face limitations in scalability, performance, or circularity. Engineering biology offers a fundamentally different approach, harnessing the power and abundance of nature to create truly sustainable and high-performing materials.
Engineering biology opens the door
Engineering biology’s revolutionary approach to fashion is both lower-impact and cruelty-free. Instead of relying on fossil fuels or animal products, innovators are harnessing the power of microorganisms — nature’s tiny engineers — to create entirely new categories of materials to service fashion and beyond. Not only do these materials look and feel beautiful, consumers can also feel proud to wear them. But how do we get from microscopic organisms to sheets of material that can be stitched into a handbag?
There is a wave of material innovation companies in the UK developing scalable solutions to meet the industry’s need for high-performing, versatile materials that don’t pollute the planet. For example, Arda Biomaterials and PACT Biomaterials take polymers from brewery and fishery waste respectively and convert them into new materials. Other companies, such as Really Clever and BIOHM, use fungi as a feedstock. Some, such as Solena Materials, are even using AI to help design completely new fibres from scratch, with potential applications not only commercially, but also for the police and the military where new fibres that are more effective at absorbing energy could create safer blast, ballistic and stab-proof gear.
One particularly interesting company is Modern Synthesis. Their process starts with microbes that naturally produce a material called nanocellulose. Nanocellulose is a fermentation-derived polymer that forms fibres mere billionths of a metre wide. These fibres can host additional ingredients to achieve new material forms, functions, and feels.
The nanocellulose can be enhanced using materials science to help transform it into a biofilm. This can be combined with a natural textile backing (such as cotton, linen or hemp) to form an innovative composite material, which is then finished with natural coatings like waxes and oils to enhance performance and fine-tune how it looks and feels. The finished materials can be used in place of a variety of textiles, from films to leathers — without needing petrochemicals or toxic ingredients, and offering a reduced environmental impact. This goes beyond current attempts to use synthetic chemistry to create greener versions of existing plastics, or ‘biosynthetics’, where biobased feedstocks are used to create identical products to petrochemical plastics.
Modern Synthesis has partnered with fashion brands to develop prototypes using these vegan and non-toxic materials. They are working towards a commercial launch of fashion accessories such as handbags in 2026, as well as developing a version of the material for footwear. Although the initial materials are being produced using naturally-occurring bacteria, the company has also experimented with modified organisms to introduce advanced properties such as self-dyeing, where pigments are produced at the same time as the nanocellulose fibres.
A diagram showing the process to turn microbes into a new material. The diagram shows number one as a petri dish with a few small colonies or spots. Then number 2 is a set of test tubes with caps. Number 3 is a microscope slide with a cover slip and a sample in the middle. Number 4 is cylindrical rolls of material, and 5 is the finished handbag.
Figure 6: A simplified schematic of the process employed by Modern Synthesis to turn naturally produced microbial products into new materials for commercial use. (1) Microbially-derived raw material (nanocellulose) can be converted into (2) a proprietary bio-based formulation. (3) This can then be integrated into textiles with structural backing to eventually produce (4) rolls of finished materials which can be used to make (5) fashion accessories free from toxins and animal inputs.
Impact
By transitioning to materials created through engineering biology, the fashion industry can significantly reduce its environmental footprint and contribute to a cleaner, healthier planet. It would also unlock economic benefits, creating new jobs in the bioeconomy without compromising on the quality, performance and aesthetics of the products we love.
Environmental benefits: These innovative materials have the potential to offer significant reductions on the greenhouse gas emissions associated with traditional material production. This includes minimising the environmental impact of raising livestock for leather and avoiding some of the energy-intensive processes involved in creating synthetic textiles. Furthermore, these materials can be designed for biodegradability, offering a solution to the pervasive problem of microplastic pollution.
Economic benefits: Engineering biology is a growing sector and one that is continuing to attract investment (Thomsen, 2024). It has the potential to create high-paying jobs in research and development, particularly when leveraging the UK’s world-leading university research and lively spinout ecosystem. Targeting engineering biology at materials production also offers the potential for high-paying, high-value manufacturing jobs. Meanwhile, UK agriculture could benefit from increased demand for biobased feedstocks, particularly where residual/waste feedstocks can be utilised to maximise value from each harvest.
Better products: Engineering biology can create biomaterials with a huge range of structures and functional properties, built from the nanoscale up. Textiles can be tuned to modify and improve features such as texture, softness, breathability, and resistance to temperature or moisture. For example, Modern Synthesis has demonstrated materials with improved UV and temperature resistance compared to synthetic plastic equivalents, meaning that they don’t discolour, crack or lose shape in the sun. Other companies in this field are producing leather-like materials that directly mimic the composition, patina, and wear of animal leathers, unlike synthetics such as polyester or polyurethane that shed, peel and crack over time.
Affordability: As the scale of manufacturing increases, these new materials are increasingly becoming cost-competitive with synthetic materials, and with support they could be cheaper in the long term. Durable, longer-lasting materials are ultimately more affordable — assuming that consumers support the regulatory push towards ending fast fashion (European Parliament, 2023). Biobased chemicals and materials are produced from abundant and widely available natural raw materials (biomass) and so are decoupled from the volatility and supply chain pinch points (American Coatings Association, 2019) of petrochemicals.
A black and white handbag, with a black flap and a patterned body. Text sections around the pouch describe its features: unique natural haptics and aesthetics, durability to light and temperature changes, biobased and zero hazardous chemicals, and pathways to recycle or safely biodegrade.
Figure 7: Some of the benefits that could be offered by new biobased materials include new feels, new functions, increased safety and improved biodegradation.
The work ahead
A bioeconomy transition powered by engineering biology presents an opportunity equivalent to the materials revolution of the 20th century, when natural polymers and fibres such as silk were replaced by petrochemically-derived synthetics like Nylon (American Chemical Society, n.d.). Once again, fashion is leading the way in the material transition.
To achieve the vision of a more sustainable fashion industry, continued investment in research and development in engineering biology will be required. Academic institutions and Catapult Centres will likely play important roles as this sector continues to grow in years to come. As is the case in other sectors of the bioeconomy, scale-up remains a significant challenge in the world of biobased materials, and that will need to be tackled if the many exciting start-ups in this space are to thrive and have maximum impact.
Regulation will also have a key role to play to enable heritage fashion and textile industries to shift to higher-value manufacturing of revolutionary new materials. Approaches will need to be forward-thinking and tackle challenges around biosafety categorisation and licensing processes for genetically-modified organisms, as well as supply chain transparency.
It’s important to note that engineering biology, and the materials innovations it empowers, is not a silver-bullet solution to fashion’s sustainability crisis. However, it is a crucial piece of the puzzle and works in tandem with other circular economy initiatives, such as sustainable sources of biobased feedstocks, scaling recycling infrastructure, and building consumer awareness.
Potential challenges and societal considerations
Cost is a significant barrier to applying engineering biology in fashion, because these bio-based materials are currently more expensive than synthetic materials. As these technologies mature and companies begin to achieve economies of scale in manufacturing, costs are expected to come down. But many of the current innovations are being produced by startups and SMEs, which will need support to scale up production themselves, or partner with existing large-scale material manufacturers.
Regulatory environments that price in or expose the true costs of environmentally-damaging synthetics could also help these technologies scale. So too could government guidance that supports domestic (or allied country) production of critical materials such as textiles. For example, the US BioPreferred Program (USDA, n.d.) mandates federal agencies and contractors to source biobased products from domestic feedstocks, where these alternatives exist. This aims to support the uptake and transition to biobased ingredients and materials, creating more resilient supply chains and reducing dependence on foreign petrochemicals.
Consumer acceptance could pose another challenge, as some consumers may be hesitant to wear clothes made with microbially-derived materials. However, as people become more aware of the environmental impact of fashion, they may become more receptive to sustainable alternatives. Unlike other engineering biology applications, materials and textiles production does not require the release of modified organisms. The production strains are removed during processing and the finished products are sterilised before entering the market. This makes these applications a potentially more acceptable public-facing demonstration of engineering biology applications.
A transformative national capability
The need for sustainable fashion is more urgent than ever. Climate change is a global crisis, and the fashion industry is a major contributor. Consumers are also increasingly demanding sustainable products. Engineering biology is now at a tipping point where it has the potential to meet those challenges and revolutionise the fashion industry.
The UK is uniquely positioned to realise these benefits, with a celebrated heritage in fashion and textile manufacturing, and a thriving creative sector. These could be coupled with our strong academic and early-stage company leadership in advanced materials and biotechnology innovation.
Successfully applying engineering biology in fashion could have a significant impact on the UK economy. Enabling and supporting fashion’s transition to the bioeconomy has the potential to reinvigorate the UK’s leather and textiles industries, supporting higher pay and economic growth across regions beyond the ‘Golden Triangle’, where biotech impact is usually concentrated. It could also encourage re-onshoring of higher-value manufacturing, shorten supply chains for critical resources, and thus improve resilience.
Finally, these opportunities align with the government’s missions of “kickstarting economic growth”, “making Britain a clean energy superpower”, and lifting up traditional regional industries to “break down barriers to opportunity”. It represents a materials revolution that the UK should be ready to lead.
2.4 Lab-Grown Blood
Creating a safe and unlimited source of blood for all.
Imagine a world where no patient dies due to a lack of compatible blood during surgery, accidents, or medical crises. It’s a world where lab-grown blood is produced in huge factories, and available on demand. The blood is engineered to be more universally compatible with a range of blood groups. It is also free of disease transmission risks, and is easier to store. Although blood services will still collect human blood, sourcing rare blood types for transfusions is no longer a challenge. The logistics of supplying safe and compatible blood is vastly improved, enabling faster responses to military or humanitarian situations, and providing a more sustainable and equitable blood supply for all.
Current status
The world relies almost entirely on human blood donations to meet medical needs such as haemorrhage, anaemia or disease. But blood transfusion services face significant limitations. In many low- and middle-income countries, there is a critical shortage of safe blood (Raykar, 2021). Even in the UK, blood donation rates can fluctuate, causing shortages that can be especially problematic during emergencies. Donated blood has a limited shelf life, making it harder to manage supplies. The logistics of sourcing, storing and distributing donated blood can be even more challenging in military or emergency situations, or in countries that lack infrastructure to deliver safe blood products.
It can be particularly difficult to source rare blood types, and very occasional mismatches in blood groups can sometimes lead to life-threatening complications (Hawksworth, 2018). And although blood services use screening processes to avoid known infections, there is always a threat of new pathogens arising, which can be passed on to transfused patients. Additionally, neonates – babies under 28 days of age – can only receive transfusions of blood that is free from cytomegalovirus (CMV). Given that the majority of adults have had CMV at some point in their life, only a small percentage of donated blood can be given to neonates, meaning that there are often short supplies for emergency transfusions.
Various synthetic blood substitutes have been developed to address these challenges. Some are based on solutions of haemoglobin, the oxygen-binding protein that gives red blood cells (RBCs) their colour. Others rely on inert liquid chemicals called perfluorochemicals, which form emulsions that can be used to carry and deliver oxygen. There are multiple ongoing clinical trials around the world using these substitutes (Tanner, 2024). While these different approaches have shown some promise, issues like limited oxygen-carrying capacity, toxicity, logistics, and high production costs have so far hindered widespread adoption.
Researchers working in the field of regenerative medicine can also grow RBCs from stem cells — so-called ‘master cells’ that have the potential to transform into many different cell types. Some of these stem cells have been harvested from adult blood, while others come from umbilical cord blood (Pellegrin, 2021). However, there is currently only one published study detailing a small transfusion of lab-grown RBCs, which were grown from the recipient’s own stem cells (Giarratana, 2011).
NHS Blood and Transplant (NHSBT) and partners are now conducting the RESTORE trial (NHS, 2022), a clinical assessment of mini-transfusions of lab-grown RBCs that should produce results by the end of 2025 or early 2026. Crucially, these cells come from volunteer donors, rather than the recipients themselves. Although groundbreaking, all these efforts have yet to achieve the scale and efficiency required for widespread clinical application.
Engineering Biology opens the door
Advances in regenerative medicine, biomanufacturing, and synthetic biology are converging to drive an engineering biology revolution in RBC production. This could solve many of the challenges with storage, logistics, and access to rare blood groups that blood services currently face.
Techniques from synthetic biology have recently been used to produce a new, potentially more sustainable, source of freshly made RBCs (Trakarnsanga, 2017). This involves taking developing blood cells from donors, and genetically reprogramming them so that they keep growing and dividing forever. Since these ‘immortalised’ cells can be grown indefinitely in culture, they could be produced in large amounts and banked for future use, potentially providing an unlimited supply of RBCs (Sivalingam, 2021).
By carefully selecting appropriate donors, and potentially using gene editing to delete multiple blood-group genes, researchers can also create ‘universal’ lab-grown RBCs that are highly compatible with a range of different blood groups (Hawksworth, 2018). Other genetic modifications can give the RBCs additional properties — expressing enzymes that treat certain diseases, for example, or that offer protective antioxidant effects (Langlands, 2024; Meinders, 2020). Scarlet Therapeutics, a spin-out from the University of Bristol and NHSBT research, is now using this approach to commercialise therapeutic RBCs.
Meanwhile, regenerative medicine studies have confirmed that lab-grown RBCs are functionally equivalent to natural, newly-produced RBCs in the body (Kupzig, 2016; Moura, 2018). Trials like RESTORE are aiming to demonstrate the clinical feasibility of these lab-grown RBCs, while biomanufacturing innovations, such as scalable bioreactors and automated production processes, are paving the way for large-scale, sustainable RBC production.
With effective investment and testing, these precious blood-producing cell lines could represent the future of transfusion medicine. Lab-grown RBCs promise a safe, scalable, and sustainable solution that is free from infection, ensuring a resilient and equitable blood supply that meets the growing global demand. This has the potential to help save countless lives, particularly in emergency medicine and surgeries, while empowering healthcare systems, reducing dependence on sourcing donors, and addressing inequalities in blood availability. Global beneficiaries include patients, healthcare providers, humanitarian organisations, and governments striving for healthcare resilience. Excitingly, a successful roll-out of lab-grown RBCs could lead to similar advances for other blood components, including engineered platelets or immune cells.
A diagram illustrating lab-grown blood production. Number 1 is an image of a donor blood bag, number 2 is the red blood cells being extracted and immortalised, number 4 is the DNA being edited, number 4 is an image of the laboratory equipment, number 5 is the lab-grown blood combining with other blood cells, number 6 is the blood being filtered, and number 7 is the blood product at the end of the process.
Figure 8: A diagram depicting a simplified pathway to producing lab-grown blood. (1) Blood originally gifted by a human donor is used to (2) grow and genetically program red blood cells to become immortalised. (3) This cell line can be genetically edited to introduce new characteristics, for example for therapeutics or to create a universal blood type. (4) These red blood cells can be produced at small-scale in laboratories up to larger-scale in reactors. (5) Lab-grown red blood cells can be combined with other constituent blood cells to create a mixed population which can be (6) filtered to produce (7) the final lab-grown blood product.
The work ahead
The convergence of technologies in synthetic biology, automation, and advanced materials makes this the right moment for investment. The COVID-19 pandemic underscored the fragility of global blood supply chains, highlighting the urgent need for innovative solutions. Furthermore, the rising global population, the challenge to motivate and secure more blood donors, and the increasing longevity of populations, will amplify the demand for reliable and scalable blood sources in the coming decades.
In addition, it remains challenging to establish a secure blood supply in military settings that covers both ends of the operational spectrum. At one end is the need to get blood products to small teams close to the point of injury when this is remote and logistically challenging. At the other end is the need to supply huge volumes of blood products in a large-scale combat operation. Both contexts necessitate development of products that are more universal, easier to store, longer lasting, and non-reliant on traditional human donation. A sustainable, more universal blood supply would provide a significant advantage to UK armed forces, and to those of partner countries.
With the recent advances made by UK scientists in this area, including the RESTORE trial, we are at a pivotal moment for the UK to lead the world in this exciting form of cell therapy (Tanner, 2024). The following steps and milestones could provide a blueprint towards achieving the vision of widespread availability of lab-grown blood in the next 10 years:
Scaling production: develop scalable blood production processes and bioreactors capable of manufacturing lab-grown red blood cells in clinically relevant volumes.
Clinical validation: conduct phase 1 and phase 2 clinical trials to establish the safety and efficacy of lab-grown blood products from cell lines or other equivalent cellular sources.
Regulatory frameworks: collaborate with regulatory bodies to create guidelines and standards for lab-grown blood production, testing, and distribution.
Potential challenges and societal considerations
There are a number of technical barriers that still need to be overcome in order to pave the way for cost-effective and large-scale production of functional RBCs. This is likely to require new types of manufacturing facilities, bioreactors, and methods for efficiently isolating end products from the manufacturing process. Robust public and private investment could help to bridge the gap between lab-scale production and commercial-scale implementation. Incentives for public-private commercial partnerships, for example between industry, military and NHS organisations, could be one way of driving capability and investment.
Another way of facilitating the scale-up process could be to create at least one specialised hub, or potentially a network of R&D hubs, equipped with state-of-the-art biomanufacturing facilities for lab-grown blood development. This would ideally be closely linked to a clinical facility, to undertake clinical studies simultaneously.
Developing the workforce for the future of this field is another challenge that is likely to require significant cross-disciplinary collaboration to integrate expertise from biology, engineering, data science, and clinical medicine. Expanding the training programs available in synthetic biology, bioengineering, and biomanufacturing will also play a key role in creating a skilled workforce.
It will be important that those developing and deploying this innovative technology engage with regulatory bodies, in order to ensure the correct processes are employed for use in humans and compliance with rigorous safety and efficacy standards. Engagement with the public will also be vital for offering reassurance on ethical concerns about lab-grown blood products and helping to foster public trust.
A transformative national capability
Developing lab-grown blood solutions is a multi-billion-pound endeavour that is likely to require sustained investment over the next decade to be successful. However, immortalised blood cells have the potential to act as a ‘platform technology’ of the future, forming the foundation for a broad range of applications in the healthcare space including new types of RBC-based therapies, and in doing so driving innovation, intellectual-property generation and economic growth. The long-term benefits that this could provide from both a healthcare and economic perspective could be considerable, delivering on the government’s missions of both creating “an NHS fit for the future” and “kickstarting economic growth”.
The UK is currently a world leader in lab-grown RBCs, pioneering both the fundamental research as well as the first steps towards application in humans through the RESTORE trial. Maintaining this position as the technology reaches commercial viability could help to create high-skilled jobs in synthetic biology, cell manufacturing and cell therapy. Establishing the UK as a leader in biomanufacturing innovation could also provide enhanced resilience against supply chain disruptions during pandemics or natural disasters, boosting healthcare security.
On an international scale, lab-grown blood could play a vital role in supporting global humanitarian efforts by providing sustainable blood supplies during emergencies. There is the potential for this technology to drive a reduction in global healthcare disparities, particularly in rural or underserved regions across the globe. This will require careful consideration of processes to support technology transfer, but represents a transformative opportunity for national healthcare systems, contributing to UN Sustainable Development Goals (SDGs), such as health (SDG 3) and reduced inequalities (SDG 10).
2.5 Microbial Metal Factories
Forging a sustainable future for the metals our industries rely on.
Imagine a world where we’ve solved metal scarcity. Engineered microbes work tirelessly to extract and recycle metals from electronic waste, so that we no longer rely on harmful mining practices. A secure, domestic supply of bio-recovered metals has reduced our dependence on imports, particularly from conflict-affected areas. These vital metals power our green energy technologies, enabling our cities to thrive. In this future, engineering biology underpins global sustainability goals, and shows that economic progress can coexist with environmental preservation.
Current status
Metals are critical for sustainable energy technologies (Dent, 2012; Smith, 2022; Valckx, 2021). For example, the batteries in electric vehicles depend on metals including lithium, cobalt and nickel. Rare-earth elements such as neodymium are essential for the powerful magnets in wind-turbine generators. And copper wires form the arteries of our increasingly electrified society.
Building the infrastructure for the energy transition hinges on a secure supply of technology-critical metals, and that is driving a global race for these indispensable resources. By 2040, nickel and cobalt demand will increase up to 25-fold, demand for the rare-earth elements will increase 7-fold (IEA, 2021) and the copper demand for energy transition technologies will triple (Cox, 2022).
Most metals are extracted from ores. These rocks contain minerals that are chemical compounds of metals. Traditional approaches to producing metals require huge amounts of energy — not only to mine the ore, but also to separate the required minerals from the rock, and free the pure metals from their chemical compounds. These processes can all generate pollution, causing severe environmental degradation and biodiversity impacts. Some mining operations also raise important ethical concerns, such as the use of child labour for cobalt mining.
There are also concerns over whether our current sources of metals will be sufficient to meet future demand (Valckx, 2021). This is causing a shift towards mining low-grade ores, despite the fact that these processes can be more energy- and resource-intensive and still produce less of a lower-quality metal. Meanwhile, attempts to increase the recycling rates of critical metals are also facing significant hurdles. Electronic products can contain up to 60 different elements (Bloodworth, 2014), so isolating each individual metal poses a major challenge for today’s chemical purification methods.
These methods are not only inefficient but also environmentally damaging, often requiring high temperatures and toxic chemicals, contributing to carbon emissions, resource scarcity and ecological harm. As a result, many electronic items are not effectively recycled and are instead ending up in landfill (Bloodworth, 2014). Without significant changes, these issues will only intensify as global demand for electronics and green technologies rises.
Engineering biology opens the door
Using innovative engineering biology techniques, we now have microorganisms that are able to efficiently extract and recycle metals from wastes (Echavarri-Bravo, 2022). Some microbes can dissolve metals from multiple sources, including low-grade mined ores and electronic waste; others can transform these dissolved metals into tiny insoluble nanoparticles that can be separated from the liquid. By pairing these processes together and enhancing the underlying biochemical pathways in the microbes, we can boost the production of nanoparticles to create an effective metal recovery process.
At the University of Edinburgh, this approach has been used to recycle metals from end-of-life automotive lithium-ion batteries, for example (Pakostova, 2024). First, the metal-containing components of the batteries are dissolved by chemical or biological methods. Then the bacteria get to work, removing the dissolved manganese, and producing cobalt and nickel nanoparticles (Echavarri-Bravo, 2022). Once these metals have been extracted, they can be used to build new batteries or used in other green technologies. Meanwhile, the remaining liquid is a lithium-rich brine, similar to those found in South American lithium deposits, which is also useful for battery production. All of the recovered metals are classed as critical minerals in the UK 2024 Criticality Assessment (Mudd, 2024), and the process has the potential to revolutionise sustainable metal extraction and recycling in the energy sector.
A process flow diagram for recovering metals from end-of-life batteries and electronic waste. Steps include: Metal Ores, Dissolution, Engineered Bacteria, Nanoparticle biosynthesis, and Recovered Metals. A feedback loop indicates the use, reuse, and remanufacture of recovered metals back into the process
Figure 9: A simplified schematic of the process that uses bacteria to recover critical metals from ores and from electronic waste, so that they can be recycled and reused.
Other microbes can mine liquid waste to generate nanoparticles of the precious metal palladium. This metal is widely used as a catalyst to speed up chemical reactions involved in making pharmaceutical drugs, for instance. The nanoparticles created by microbes could actually offer an improvement on traditional palladium catalysts, not least because they work at lower temperatures and in water (Era, 2021; Era, 2022). This could reduce the need for petrochemical solvents and acids in these chemical reactions, helping to make industrial processes more sustainable.
Silver and copper nanoparticles can be produced in similar ways. Tweaking the living conditions and engineering the microbes can fine-tune the size, shape and stability of the nanoparticles they produce — which could be used to create robust anti-microbial coatings with less metal than conventional approaches, for example (Pantidos, 2018; Ramanathan, 2011).
By avoiding hazardous chemicals and reducing our reliance on finite natural resources, these bio-based methods support a circular economy for technology-critical metals. As global demand surges, microbial metal factories present a huge opportunity for sustainable growth. They not only offer economic advantages by reducing costs and resource dependency but also contribute to achieving broader environmental and societal goals.
The work ahead
To realise the full potential of this emerging technology, we need to deepen our fundamental knowledge of exactly how microbes gather these metals and turn them into nanoparticles. This foundational research can then be translated into practical advances in engineering biology — honing each microbe’s preference for a particular metal, increasing their output of nanoparticles, and tailoring the properties of those nanoparticles to their future function.
Engineering biologists can use these methods to produce novel materials with exciting new properties (Echavarri-Bravo, 2025). Research has already demonstrated that biosynthesised metal nanoparticles offer improved catalytic efficiencies (Era, 2022) and increased stability (Pantidos, 2018), enabling various industrial processes to use less material without sacrificing performance — a true circular economy intervention. Meanwhile, we can also use artificial intelligence (AI) to help identify the best conditions for the bio-recovery of metals, minimising our experimental requirements and dramatically accelerating our journey to scale up these methods.
Technology providers are yet to be convinced of the performance of bio-recovered metals, so engineering biologists will need to collaborate with them to understand how these materials might be incorporated into products. The large-scale recovery of metals using engineering biology also presents specialised scale up challenges, and may require systems akin to wastewater treatment. Consequently, collaboration with waste management sectors will be essential to integrate these bio-processes into existing recycling systems.
We also need to be sure that bio-recovered metals offer genuine environmental benefits. That will require life-cycle assessments that carefully track the fate of materials in these systems, their energy use, and their environmental emissions. Those assessments should be integrated into engineering biology efforts at a relatively early stage, in order to direct research developments to provide the best sustainability gains.
Investment in research and development, training a skilled workforce, and building infrastructure such as bioreactor facilities, will all be necessary to scale up these methods. Pilot programs in industrial settings will test and refine processes, moving from the lab to market through public-private partnerships. These steps will ensure a smooth transition and foster collaboration across sectors.
Potential timeline milestones
2025 to 2030: Laboratory-scale experiments to optimise microbial strains for specific metal extractions, exploiting national capabilities in DNA construction, strain testing and AI-assisted learning. Projects should be interdisciplinary, collaborative (nationally and internationally) and co-created with recycling facilities and mining companies, to focus on integrating microbial processes into existing systems.
2030 to 2035: Scale-up operations at advanced bioreactor facilities, navigating regulatory approval processes. Continued development of interdisciplinary partnerships with waste management and mining companies to create hybrid systems that combine biological and traditional methods.
2035 to 2040: Full-scale deployment and commercialisation of sustainable mining and microbial recycling technologies, with widespread global adoption.
Potential challenges and societal considerations
Despite the promise of microbial metal recovery, it also poses challenges and risks. Engineered microbes must be properly contained to avoid any potential ecological impacts, for example. Work will also need to continue to understand how best to safely manage, and chemical or material challenges associated with these processes. However, any risks should be contrasted with the significant environmental hazards already posed by current and historical mining sites, along with metal processing and recycling practices that continue to contaminate land and deplete biodiversity.
Nevertheless, public apprehension about genetically modified organisms, whether founded or unfounded, might pose a barrier to their wider use for metal recovery. Addressing these issues will require robust safety measures, stringent containment protocols, and transparent communication that builds trust. The practical steps needed to alleviate concerns and build enthusiasm about this revolutionary technology will strongly influence overall cost.
If successful, this technology will transform even the most complex forms of metal-bearing waste into a valuable resource. In doing so, it could change how society views both current and historical waste, which might lead to risks like mining of landfills without the required environmental safeguards and unregistered waste handling. Tackling that may require updated waste regulations, revised terminology, and redefining waste classifications.
The financial costs of developing bio-recovery systems for metals will be significant. Private investment may be sufficient for the most valuable and critical metals, but sustained public investment will also be required to capitalise on the full breadth of the opportunity. The development and deployment phases would likely span decades, requiring ongoing support and collaboration. It will involve technoeconomic analysis to compare the relative merits of small-scale, widely distributed and perhaps more specialised metal recovery facilities, with larger sites that involve many metals and multiple industries. This will depend on factors such as capital expenditure, transportation requirements, environmental impact, and health and safety risk-mitigation.
The long-term economic and environmental benefits of this technology have the potential to outweigh initial investments, resulting in reduced dependence on raw materials and widespread environmental remediation.
A transformative national capability
Adopting microbial metal recovery has the potential to create jobs across all skill levels. It could benefit former mining areas by providing low-cost and sustainable tools to access remaining low-grade ores. This approach could also financially incentivise the remediation of mine tailings, limiting their pollution and environmental damage. And by enabling more efficient recycling practices, it could support an expansion of the recycling industry.
Nationally, microbe-driven recycling would also reduce our reliance on imported metals, strengthening economic security and providing resilience to sudden price fluctuations caused by extreme events, be they political or environmental. Industrial sectors reliant on technology-critical metals — such as manufacturing, transport and energy — could benefit from a more consistent, eco-friendly supply, while significantly lowering their environmental impact. The technology aligns with the UK’s Critical Minerals Strategy, as well as the country’s ambitions to advance clean energy infrastructures and national sustainability targets. Ultimately, this application of engineering biology could help to lower carbon emissions and build a circular economy in the UK, delivering on the government’s mission to “make Britain a clean energy superpower”.
Developing this technology to its greatest potential could have an even bigger impact on global politics, because conflicts are so often motivated by access to resources such as minerals. Integrating biotechnology into metal recovery processes would allow us to address some of these global resource demands.
Innovation in this area complements government strategies focused on fostering international collaboration and technological exchange. It would support global sustainability goals, contribute to action on climate change, and help to achieve a global circular economy in which resources are conserved, ecosystems are protected, and economic growth is decoupled from environmental harm. By reducing pollution and ensuring supplies of ethically-sourced metals, engineering biology will help enable a just transition to a sustainable future.
3. Challenges for the future
In this concluding chapter, we have tried to encapsulate what a future world might look like if we are successful in exploiting the full potential of EngBio in addition to identifying some of the potential challenges to achieving a positive outcome. To do this, we have created an optimistic and a pessimistic vision for the future of EngBio. These visions are intended to stimulate thinking around how we can seize the opportunities offered by EngBio and minimise or mitigate the potential downsides. They are highly speculative and represent 2 extremes of what the future of EngBio might look like — they are not predictions.
To create the visions, we combined key points from the aspiration papers with the outputs from a series of interviews that we held with CSAs, including the GCSA, on what they thought the future might look like for EngBio in the coming 10 to 15 years. The discussions were conducted using the 7 Questions (7Qs) futures methodology (Government Office for Science, 2024). Originally developed by Shell to support their strategy development process, 7Qs is an interview technique for gathering the insights of a range of stakeholders about the future. A summary of the output from the 7-Questions discussions is provided in Appendix A.
An optimistic vision for the future
There are clearly huge opportunities for EngBio to solve real-world problems and have a positive impact on people and the planet: by creating new jobs and economic growth, by offering more sustainable ways to provide the goods and services we rely on, and by improving UK prosperity and national security. A positive but realistic vision for the future of EngBio might therefore look something like this:
Imagine a world where engineering biology (EngBio) has revolutionised our world. In this scenario, EngBio powers a thriving, modern manufacturing industry that produces innovative, sustainable products and services, creating countless jobs and vibrant communities. The UK, by focusing on cutting-edge areas, remains a global leader in specialised EngBio applications, driving economic growth and technological advancements.
Our food system is transformed through precision breeding and other EngBio technologies, resulting in healthier, more nutritious food that can grow in a changing, challenging climate. Environmental challenges are addressed with EngBio solutions that safely produce essential goods without harmful byproducts, contributing to a cleaner, greener planet and achieving net-zero emissions.
International collaboration flourishes, accelerating the development and application of EngBio innovations worldwide. This global effort enhances our collective ability to tackle pressing issues, from climate change to public health.
EngBio also strengthens our security, providing advanced vaccines, therapeutics, and diagnostics to protect against future pandemics and bioweapons.
In this optimistic future, EngBio not only improves our quality of life but also ensures a sustainable, secure, and prosperous world for generations to come.
A pessimistic vision for the future of EngBio
However, the future is rarely, if ever, as optimistic as we hope and as with any technology, there will be challenges that will need to be overcome if we are to achieve our desired outcome. To understand what the barriers to achieving our positive vision might be, it helps if we challenge ourselves by thinking about what could go wrong and what a less positive future might look like.
In a pessimistic future, the UK fails to establish its niche in EngBio, falling behind other countries that develop superior EngBio ecosystems more rapidly. This results in the UK becoming reliant on importing EngBio products and services, leading to a loss of strategic advantage and missed economic growth opportunities.
The potential for malicious or accidental harms becomes a reality, with ‘bad actors’ deliberately releasing EngBio-enabled pathogens or accidental environmental impacts causing public backlash and eroding trust in EngBio technologies.
Promising EngBio applications fail to meet expectations, proving too complex or uneconomical to scale, leading to public disillusionment, investor disappointment, and inconsistent adoption of EngBio solutions. Unintended outcomes, such as resource competition over new feedstocks, further exacerbate the challenges, creating new areas of conflict and hindering progress.
This bleak scenario highlights the risks and obstacles that could undermine the potential benefits of EngBio.
What is already underway
As outlined in Chapter 1, the National Vision for Engineering Biology outlines the government’s ambition for EngBio by providing a framework for the future direction of government investment, policy and regulation (Department for Science, Innovation and Technology, 2023b). Government has made progress delivering on the commitments made in the National Vision, for instance, UKRI’s announcement in February 2024 of a new £100 million fund to unlock the potential engineering biology (UK Research and Innovation, 2024). However, what is clear from the 5 case studies is that there are some challenges and barriers to achieving a positive outcome, several of which are complex and cut across almost all sectors and applications.
Remaining challenges
The following are some of the key issues highlighted by our expert authors and the visions for the future outlined above. These need to be tackled in years to come if we are to harness the full potential of engineering biology. This is by no means a comprehensive list and each specific application of this technology will likely have several sector-specific challenges to commercialisation. Nevertheless, there were several recurring themes across Chapter 2 which we aim to summarise below, to convey to readers the nature, scale and importance of each challenge, as well as signposting to recent and ongoing work in the UK and beyond.
1. Scaling-up and funding
In order for EngBio to effectively address some of the substantial global challenges referenced throughout this report – for example, sustainable production of fuels, materials, and food – it is essential that there are clear routes in place for translating research and development from the laboratory scale through to the industrial scale and commercialisation. This process is referred to as ‘scaling up’ and typically requires the use of infrastructure such as laboratories, production plants, and biorefineries.
There are several key parameters to consider as part of the scaling-up process, including costs, regulation, supply chains, location, and the nature and size of the market being targeted.
Lack of funding is many times a root cause for lack of scale up. All aspiration papers highlight the need for investment to ensure that they have facilities with enough specialised equipment to achieve success on a commercial scale. This investment needs to be sustainable for consecutive years to successfully bring products into the market, by maintaining progress and reaching new levels of innovation. The aspiration papers also draw out that not only will EB bring out societal benefits in the respective sectors, but it also has the potential to drive economic growth. For example, in the aspiration papers “Lab-Grown Blood” and “Microbial Metal factories”, it is expressed that the long-term economic benefits will outweigh the initial investments.
The UK government understands the need for increased funding and infrastructure. The National Vision for Engineering Biology (Department for Science, Innovation and Technology, 2023b) addressed the concerns around SMEs requiring well-equipped facilities and more funding. To reach the goals described in the National Vision, 22 Mission Awards were funded as well as 6 EngBio Mission Hubs. The hubs will receive up to £12 million each over the course of 5 years. This funding showcases the importance of EngBio to the UK and emphasises the potential of EngBio to tackle challenging issues in society (UK Research and Innovation, 2024).
2. Public Perception
Four out of the 5 aspiration papers suggest that public perceptions can create a barrier to the acceptance of EngBio in those fields. Both the ‘Nitrogen-Fixing Cereals’ and ‘Microbial Metal Factories’ papers highlight the possibility that negative public opinion on genetically modified crops and organisms may hinder the progress of EngBio in sustainably growing crops or extracting metals. The idea of negative perception is also prevalent in the ‘Lab-grown Blood’ paper, as the public may question the ethics of lab-grown products.
These views correlate with the findings in reports published by Sciencewise which focussed on public perception of EngBio. The reports studied public perception on 2 major application areas, food (Sciencewise, 2024a) and health (Sciencewise, 2024b). The report on EngBio being used in the food industry highlighted that people are concerned about the “unnaturalness” of GM crops. Similarly, the report on the health application also showed people’s concerns on blurring the boundaries between natural and artificial medicine. However, the public did express optimism when EngBio is utilised to tackle a specific and clear issue.
We know work is needed to engage with the public as early as possible in the development of these technologies, to communicate how they work and how they could change our lives for the better but also hear from the public about their needs and concerns. The GM crops legacy shows us where we can go wrong, but we also have examples where we have got this right.
The Genetic Technology (Precision Breeding) Act 2023 (UK Parliament, 2023) sets out a framework for the governance of precision bred organisms including crops and animals. It was informed by an ethical review and public dialogue undertaken by the Nuffield Council on Bioethics, which utilised ethical analysis to identify and explore the priority issues of public interest. What this showed was that the public were most concerned about how the technology would be used, for what purpose, and in whose interests, rather than the nature of the technology itself. The resultant Bill contained a provision for scrutiny by a welfare advisory body and requirement for breeders to submit a declaration that the health of the animal or welfare of the animal will not be harmed by its precision bred traits. These inclusions reflect societal values and help ensure the use of the technology can garner public trust.
In 2024, DSIT conducted a survey in which public perceptions and attitudes on EngBio were gathered and published. This stemmed from their commitment to developing insights on how the public views EngBio (Department for Science, Innovation and Technology, 2024a).
The survey identified that there was a widely held belief that EngBio will have a positive impact on science over the next 10 years, after respondents were told about possible applications. However, relatively few could describe what EngBio is without prompting, suggesting that there is a gap in knowledge among the public.
Earning public trust and maintaining a positive public perception in EngBio is crucial for taking the next steps in this field and could lead to a promising future in which EngBio is at the heart of various sectors.
3. Regulation
Businesses need to have a good understanding of current and prospective regulatory processes and know the true costs of going through those processes. Lack of understanding can negatively impact businesses and close opportunities in the field of EngBio. Development of innovative products can potentially be hindered due to difficulty in getting regulatory approval. Having a supportive regulatory environment is therefore essential if businesses are to be able to develop and implement Engbio solutions in a timely and cost-effective way.
To help provide this supportive environment, DSIT introduced the Engineering Biology Sandbox Fund to award funding to regulators to run EngBio sandboxes. This fund aims to deliver a regulatory system that preserves safety, promotes innovation and accelerates regulatory reform to encourage business innovation and investment (Department for Science, Innovation and Technology, 2024b). Round 1 winners were the Food Standards Agency (FSA) who have launched a pioneering regulatory programme for cell-cultivated products that are safe for consumers before they’re sold, whilst supporting innovation in the sector. Through the programme, the FSA is committed to completing the full safety assessment of 2 cell cultivated products within the next 2 years (Food Standards Agency, 2025). Round 2 of the Engineering Biology Sandbox Fund opened in April 2025 (Department for Science, Innovation and Technology, 2024b ).
As well as the funds, the Regulatory Innovation Office (RIO) was established in October 2024 (Department for Science, Innovation and Technology, 2024c). This office sits within DSIT, and its function is to streamline the regulatory process, making it easier for businesses to bring innovative products and services to market. By reducing red tape, the office will help fast-track approvals and ensure that different regulatory bodies work together efficiently.
4. Fundamental research, skills and training
Building a strong talent pipeline from schools and promoting lifelong learning is crucial to ensure a steady flow of trained professionals with interdisciplinary skills needed for the future of EngBio. We also need well-funded universities and research together with mechanisms and strategies to accelerate the transition of EngBio from research and development (R&D) phases into practical, real-world applications. Without the underlying theory and knowledge, it is impossible to drive forward research and development projects into industrial practice. These key points are supported by most of the aspiration papers which highlight that expanding R&D funding and training programs is crucial to create a skilled workforce and foster innovation.
Conclusion
In conclusion, the potential of EngBio to revolutionise various sectors is immense, offering solutions to some of the most pressing global challenges. The aspiration papers highlight the transformative benefits of EngBio, from sustainable production methods to economic growth and national security. However, realising these benefits requires overcoming significant challenges, including scaling up, securing long-term funding, addressing public perceptions, navigating regulatory landscapes, and fostering fundamental research and skills development. The UK’s commitment to supporting EngBio through initiatives like the National Vision, Mission Awards, and the Engineering Biology Sandbox Fund underscores the importance of this field and its potential to drive innovation and societal progress.
Moving forward, it is crucial to maintain a collaborative approach, engaging stakeholders across academia, industry, government and the public to address the barriers identified. By fostering a supportive regulatory environment, investing in infrastructure and training, and effectively communicating with the public about the benefits of EngBio, we can unlock the full potential of this technology. The journey ahead is challenging, but with sustained effort and investment, EngBio can become a cornerstone of a sustainable and prosperous future, driving advancements that benefit both people and the planet.
Appendix A: The future of engineering biology
7 Questions
In this appendix we identify some of the issues that will be relevant to the future of EngBio using the 7 Questions (7Qs) futures methodology (Government Office for Science, 2024). The intention is to provide strategic insights to help stimulate further thinking around the future of EngBio.
A series of colourful arrows pointing to the right, each labelled with a different topic and corresponding questions. Topics include: Time-traveller, Optimistic Outcome, Pessimistic Outcome, Internal Situation, Looking Back, Looking Forward, and Epitaph. Each topic explores aspects of envisioning and achieving success in engineering biology.
Figure 10: 7 Questions
Originally developed by Shell to support their strategy development process, 7Qs is an interview technique for gathering the insights of a range of stakeholders about the future. The open-ended questions provide a good way to gather opinions on strategic issues from people with a broad view of subject and related issues (Government Office for Science, 2018). Figure 10 provides a list of the 7 questions. In this case we interviewed the Government Chief Scientific Adviser (GCSA) and Chief Scientific Advisers (CSAs) from Ministry of Defence (MoD), Department for Energy, Security and Net Zero (DESNZ), Department for Environment, Food and Rural Affairs (Defra), Department for Health and Social Care (DHSC) and Office of the Chief Scientific Adviser for National Security (OCSA). The following are summaries of their responses.
Question 1
Time traveller: If you could speak to someone from the future who could tell you anything about EngBio, what would you like to ask? What would you identify as the critical issue for the future?
- Making people care about EngBio:It is not always clear to people at the moment what EngBio could do for them, even though many of the EngBio innovations we are seeing offer improved sustainability or less pollution, for example, than the current status quo. Being able to demonstrate that EngBio works as it should do, along with effective communication of the benefits and problem-solving potential of EngBio, will play an important part in raising public awareness of the potential offered by EngBio.
- Public trust and acceptance: Robust regulation and equitable implementation will be critical to ensuring that EngBio is accepted as something to deliver benefit, rather than something that makes the world less safe. Regulatory frameworks need to balance innovation with public safety in order to foster trust and acceptance.
- Integration into existing processes: Scaling up and incorporating EngBio into current manufacturing and chemical synthesis processes can be complex, involving technical barriers, resource constraints (including skills, knowledge, funding), and ethical boundaries. Overcoming these constraints will need interdisciplinary collaboration, investment in education and training, and ensuring ethical considerations guide technological development.
Question 2
An optimistic outcome: If things went well, being optimistic but realistic, talk about what you would see as a desirable outcome. What is your vision for success?
- A modern manufacturing industry powered by EngBio: In the UK and globally, EngBio could be used to create new products and services to meet our needs, resulting in a modern manufacturing sector that provides improvements in current practices, as well as creating jobs and prosperity.
- The UK focuses on specific areas to stay at the forefront: We should be selective about the areas of EngBio that the UK focuses on, identifying areas at the cutting edge where we could remain at the forefront. In particular, we should think carefully about the products and services we could specialise in.
- EngBio opens the door to a more sustainable food system: The use of EngBio-based technologies such as precision breeding could be used to drive a better food system by reducing environmental impact of farming and increasing nutritional value of the food we eat.
- Solving environmental problems with EngBio: We should capitalise on the potential for EngBio to offer safe and reproducible methods for producing drugs, chemicals, fuels, food, and drink without generating toxic byproducts or causing environmental harm when interacting with natural biology.
- International collaboration on EngBio: A positive outcome would be stronger collaboration with our international partners to better leverage resources to accelerate the transition of new EngBio from research and development (R&D) phases into practical, real-world applications.
- EngBio for greater security: Another pandemic and malicious bioweapons are among the future risks faced for the UK. EngBio advances could help mitigate these risks through the development of novel vaccines, therapeutics and diagnostics.
Question 3
A pessimistic outcome: How could the environment change to make things more difficult? What are the dangers of not achieving your vision? What factors would you worry about?
- The UK falls behind: The UK could fail to build its niche, leading to the UK being outcompeted by other countries, who build a better engineering biology ecosystem more quickly than we can. Ultimately this could lead to the UK having to import EngBio products and services from other nations, resulting in loss of strategic advantage and missed economic growth opportunities.
- Malicious or accidental harms: Nearly all technologies have the potential to be used for good or bad and EngBio is no exception. For instance, the deliberate production and release of EngBio-enabled pathogens by ‘bad actors’, or if an EngBio process accidently has a detrimental impact on the environment, resulting in public backlash and a loss of public trust and acceptance. It is to counter these possible risks that we need effective regulation.
- EngBio fails to live up to expectations: Promising applications of EngBio end up not working as expected; or they prove to be too biologically complex to produce economically at scale; or it is difficult to integrate them into existing processes. This could lead to public disillusionment, investor disappointment, and mixed uptake of EngBio solutions.
- Unintended outcomes: There may also be unintended risks. For instance, the ability to utilise new feedstocks would be good, but if those feedstocks became contested then it may open up a whole new area of resource competition.
Question 4
The internal situation: What needs to change (systems, relationships, decision making processes, culture for example) if your vision is to be realised?
- We need careful regulation: We need to make sure regulations are adaptive and fit for purpose. They’re going to be different to what we have in place currently and governments will need to adapt to ensure that we can innovate towards the best outcomes for people and the planet. The aim of regulation should be to give people enough freedom and flexibility to be creative, but with the safeguards in place to prevent harm. We also need to ensure that regulation keeps pace with the technology.
- The right kind of manufacturing capability: EngBio products and processes need to be economically viable so that companies want to produce them and consumers, such as the NHS, are able to afford them. Not only have products got to be affordable and scalable, but they have also got to be implementable, as they may have to integrate with existing process. In the case of healthcare organisations, this may involve fitting into existing care pathways or, at least, not disrupt existing pathways too much.
- The role of government: The right skills, and the acceptance that EngBio is a cross-government capability requiring a joined-up approach, are important to achieving a successful outcome for EngBio. Government also has a role to play in messaging to the wider public where EngBio can be used for good (and potentially for harm), as well as facilitating public discourse and consultation about what is ethical to do and what are the boundaries.
- Taking a whole systems approach: The areas of skills, scale-up finance, procurement, international collaborations, physical and digital infrastructure, and regulations and standards are all particularly pertinent to EngBio. It is vital that we consider these factors as part of the system as a whole — they all need to be aligned and working well if we are to grow EngBio into a thriving industry in a timely manner.
- Ensuring we have the right skills: Building a strong talent pipeline from schools and promoting lifelong learning is crucial to ensure a steady flow of trained professionals with interdisciplinary skills needed for the future of EngBio.
Question 5
Looking back: How did we get to where we are today? What are the successes we can build on? What can we learn from things that didn’t go so well?
- GM foods and the challenges of public perception: In terms of lessons we can learn, the public backlash to genetically modified (GM) foods, dating back to the 1980s, is an obvious one. The example of GM foods highlights how engaging the public in scientific advancements, and gaining their trust, has often been challenging. However, the passing of the Precision Breeding Act 2023 (UK Parliament, 2023) without the same level of public backlash, indicates a shift in public perception when it comes to genetic modification. The example of GM foods shows how important it is to listen to the concerns of the public and ensure that they understand that the benefits of these technologies outweigh the risks.
- The pace of change: The last half-century has seen rapid progress in the technologies that make up EngBio. Genetically modified organisms (GMOs) have been around for 30 or 40 years, but gene editing techniques like CRISPR are only 10 to 15 years old and have already revolutionised the field. With recent advances in supporting technologies such as AI, this pace of change is likely to continue and possibly even escalate going forwards. So far, successes have mostly been in human health, and we have yet to fully embrace the technology in the fields of animal and plant health and food production. We also have not yet harnessed EngBio in the world of materials and chemicals as much as we might have done. So, these are likely to be the future growth areas for EngBio.
- Genomics: The UK’s approach to genomics is a good example of what has worked well in the past. The UK has excelled in genomics due to strong thought leadership, collaboration between academics and industry, successful UK companies in sequencing technology, and large-scale, coordinated investments from major research funders including the Wellcome Sanger Institute. This collective effort has driven significant advancements in the field.
Question 6
Looking forward: What decisions need to be made in the near term to achieve the desired long-term outcome? What needs to be done now to ensure your vision becomes a reality?
- Scale up: We need to address the scaling-up issue in the UK. We have seen that countries, such as Belgium, are starting to pull ahead of us due to their fantastic BioBase scale-up facility (Bio Base Europe, 2025). And on top of that, they have a mechanism in place to allow people to pay to use their scale-up facility. The DSIT Engineering Biology Advisory Panel (Gov.uk, 2024) is acutely aware of this challenge, but it is not yet clear who will solve it, or what the solution is.
- Leadership: We need to show global leadership. As well as being globally competitive, we also need to work with partners to ensure we’re all ‘on the same page’ about what is safe and what isn’t.
Question 7
The epitaph: If you had absolute authority and could do anything, what else would you wish to do to achieve your vision? What would you want to be remembered for?
- We need to build a smooth runway for EngBio to take off in the UK: To do this, we need to make full use of all of the science capability that exists within the public sector to drive forward EngBio through existing frameworks such as the Science and Technology Framework, the National Vision and the recently established Regulatory Innovation Office, which is working to put in place proportionate regulation for this field.
- The Industrial Strategy: It will be vital to make sure that the Industrial Strategy takes into account the massive impact that EngBio can have across digital, technology, life sciences, high-value manufacturing and growth more broadly.
- Funding: We need well-funded universities, and research together with the mechanisms and strategies to pull Engbio through from early research to practical application. This needs to be done in a way that is safe and acceptable to society.
Appendix B: Acknowledgements
The Government Office for Science (GOS) would like to thank everyone who contributed to the work of this project and generously provided their advice and guidance.
This project was led by Martin Glasspool, Ellie Batteux and Emily Connolly. The GOS team included Rory Saitch, Eve Cox, Tasfia Khan and Georgia Cairns.
We are grateful to the individuals and organisations listed below for their time, effort, and invaluable contributions.
-
Aspiration paper authors: Prof. Louise Horsfall (University of Edinburgh), Jen Keane and Dr Ben Reeve (Modern Synthesis), Prof. Philip Poole (University of Oxford), Prof. Nigel Scrutton (University of Manchester) and Prof. Ash Toye (University of Bristol).
-
Government Departments who contributed to reviewing the project outputs and the 7 Questions exercise: Defence Science and Technology Laboratory (DSTL), Department for Business and Trade (DBT), Department for Culture, Media and Sport (DCMS), Department for Energy, Security and Net Zero (DESNZ), Department for Environment, Food and Rural Affairs (Defra), Department for Health and Social Care (DHSC), Department for Science, Innovation and Technology (DSIT), Ministry of Defence (MoD), Office of the Chief Scientific Adviser for National Security (OCSA).
-
External experts who helped to scope the project: Dr Amanda Collis (BBSRC), Prof. Tim Dafforn (University of Birmingham), Prof. Tom Ellis (Imperial College London), Prof. Paul Freemont (Imperial College London), Prof. Anne Osbourn (John Innes Centre), Dr Neil Parry (Unilever), Prof. Susan Rosser (University of Edinburgh), Dr Jay Stone (Nuffield Council on Bioethics).
-
Dr Mark Peplow for editing the report.
Glossary
Term | Definition |
---|---|
Artificial Intelligence | Technology that enables computers and machines to simulate human learning |
Bioeconomy | The use of renewable biological resources to deliver products, processes and services |
Biofilm | A sticky layer on a solid surface that contains bacteria and other microorganisms |
Bioengineering | Changing the characteristics of an organism by manipulating its genetic material |
Bioinformatics | The science of collecting and analysing complex biological data such as genetic codes |
Biorefinery | A refinery that converts biomass into fuels and chemicals |
Biomanufacturing | The process of using living systems to produce molecules and materials |
Bioprocess engineering | A specialised field within chemical engineering that focuses on the design and development of processes that utilise biological materials and organisms to produce valuable products |
Bioreactor | Vessels used to culture cells or microorganisms under tightly controlled conditions to provide optimal productivity and efficiency |
Bio-synthetic fuel | A type of renewable fuel created using biological processes |
CRISPR | A gene-editing technology based on bacterial self-defence systems which allows precise modifications to DNA sequences |
Critical minerals | Natural resources essential to produce key technologies, or to support vital industries and infrastructure, often with limited supply or geopolitical concerns |
DNA | A molecule that contains genetic information for the development and function of an organism |
DNA sequencing | A laboratory technique that determines the order of the chemical bases in DNA |
Electronic waste (e-waste) | Discarded electrical or electronic devices, such as computers, phones, and televisions, that are no longer in use or have reached the end of their useful life |
Energy transition | The process of shifting from fossil fuels to renewable energy sources |
Eutrophication | A process that occurs when a body of water becomes enriched with nutrients, which leads to excessive plant and algae growth |
Feedstock | Raw materials used as inputs in an industrial process to produce useful products. Engineering biology can seek to use alternative feedstocks to traditional fossil-fuel-based chemical processes |
Gene cloning | A molecular biology technique used to create copies of a specific gene or DNA segment |
Gene editing | Alteration of the genetic material of a living organism by inserting, replacing, or deleting a DNA sequence, with the aim of improving some characteristic of a crop or farm animal, or correcting a genetic disorder |
Genetically modified organism (GMO) | A plant, animal, or microorganism that has had its DNA altered through genetic engineering |
Halomonas | A species of bacteria which are halophilic (salt – tolerating bacteria) |
Immortalised cells | Cells that have been genetically altered to proliferate indefinitely, bypassing normal cellular aging and death processes, often used in research and biotechnology |
Lithium-ion battery | A rechargeable battery that uses lithium ions to store and release energy |
Metabolic engineering | Involves the modification of an organism’s metabolic processes to enhance the production of certain substances or to enable the organism to perform new functions |
Microfluidics | The science and technology of manipulating and controlling fluids, typically in the range of microlitres (one-millionth of a litre) to picolitres (one-trillionth of a litre), within networks of channels that have dimensions of tens to hundreds of micrometres |
Microplastic | Tiny plastic particles, typically smaller than 5 mm, that result from the breakdown of larger plastic items or are intentionally manufactured for use in products like cosmetics and cleaning agents |
Nanocellulose | Bio-based material derived from plant fibres, consisting of cellulose at the nanoscale, known for its strength, lightweight properties, and potential uses in a wide range of applications |
Nanoparticle | A microscopic particle with at least one dimension less than 100 nanometres, exhibiting unique properties due to its small size and large collective surface area |
Nanoscale | Size range of 1 to 100 nanometres, where materials exhibit unique physical, chemical, and biological properties due to their small size and large surface area relative to their volume |
Nitrogen fixation | Process by which certain bacteria or industrial processes convert atmospheric nitrogen (N₂) into forms like ammonia (NH₃) that plants can use for growth |
Nitrogenase | An enzyme complex responsible for catalysing the conversion of atmospheric nitrogen (N₂) into ammonia (NH₃) during biological nitrogen fixation |
Perfluorochemicals | A group of synthetic chemicals that contain carbon-fluorine bonds, known for their water- and oil-repellent properties, but are persistent in the environment and can pose health risks |
Platform technology | A foundational technology or framework that enables the development and integration of various applications, products, or services, often serving as a base for innovation and scalability |
Polymerase Chain Reaction (PCR) | A widely used laboratory technique in molecular biology to amplify (make many copies of) specific DNA sequences or genes |
Precision breeding | Using advanced techniques, such as gene editing, to develop plants or animals with desirable traits, accelerating the methods of traditional breeding |
Proteomics | The study of the structure and function of proteins, including the way they work and interact with each other inside cells |
Rare-earth element | A group of chemically similar elements essential to produce high-tech devices, renewable energy technologies, and electronics |
Regenerative medicine | A field of medicine that aims to restore function to damaged or diseased tissues, organs, or cells |
Regulatory sandbox | Allows businesses to test and experiment with new and innovative products, services or businesses under supervision of a regulator for a limited period of time |
Root nodule | A small swelling on plant roots that houses nitrogen-fixing bacteria, which convert atmospheric nitrogen into a form usable by the plant |
Rhizobia | A group of nitrogen-fixing bacteria that form symbiotic relationships with leguminous plants, helping them convert atmospheric nitrogen into a form that the plants can use for growth |
Rhizosphere | The region of soil surrounding a plant’s roots, where complex interactions occur between the plant, microorganisms, and the soil environment |
Stem cells | Undifferentiated cells with the ability to develop into various specialised cell types, and which have the potential to regenerate damaged tissues or organs |
Sustainable aviation fuels | A form of biofuel that powers an aircraft and leaves a smaller carbon footprint in comparison to conventional aviation fuels |
Symbiotic relationship | A close and long-term biological interaction between 2 different biological organisms. These relationships can be beneficial, neutral, or harmful to one or both organisms involved |
Synthetic DNA | Refers to DNA that is artificially created in a laboratory rather than being extracted from a natural source |
Synthetic biology | The design and construction of novel, artificial biological organisms or pathways, or the redesign and altering of natural biological systems |
Synthetic symbiosis | The artificial creation or manipulation of beneficial relationships between different organisms, often through genetic engineering or biotechnology, to achieve specific outcomes or functions |
References
Amer, M. et al (2020a). ‘Renewable and tuneable bio-LPG blends derived from amino acids’. Biotechnology for Biofuels, 13(1). doi: https://doi.org/10.1186/s13068-020-01766-0
Amer, M. et al (2020b). ‘Low carbon strategies for sustainable bio-alkane gas production and renewable energy’. Energy & Environmental Science, 13(6), pp.1818–1831. doi: https://doi.org/10.1039/d0ee00095g
American Chemical Society (n.d.). Wallace Carothers and the Development of Nylon - Landmark. [online] Available at: https://www.acs.org/education/whatischemistry/landmarks/carotherspolymers.html [Accessed: 11 February 2025]
American Coatings Association (2019). Interest in Biobased Raw Materials Alive and Well Despite Lower Petchem Prices. [online] Available at: https://www.paint.org/coatingstech-magazine/articles/interest-biobased-raw-materials-alive-well-despite-lower-petchem-prices/ [Accessed: 11 February 2025]
Bio Base Europe Pilot Plant (2025). Home page. [online] Available at: https://www.bbeu.org [Accessed: 3 March 2025].
Bloodworth, A. (2014). ‘Resources: Track flows to manage technology-metal supply’. Nature 505, 19–20. doi: https://doi.org/10.1038/505019a
Burén S. et al (2017). ‘Formation of Nitrogenase NifDK Tetramers in the Mitochondria of Saccharomyces cerevisiae’. ACS Synthetic Biology 6, pp. 1043-1055. doi: https://doi.org/10.1021/acssynbio.6b00371
Council for Science and Technology (2023). Advice on engineering biology. [online] Available at: https://www.gov.uk/government/publications/advice-on-engineering-biology [Accessed: 19 February 2025]
Cox, B. et al (2022). ‘The mining industry as a net beneficiary of a global tax on carbon emissions’. Commun. Earth Environ 3, 17. doi: https://doi.org/10.1038/s43247-022-00346-4
Cuiffo, M. et al (2021) ‘Thermochemical degradation of cotton fabric under mild conditions’, Fashion and Textiles, 8(1). doi: https://doi.org/10.1186/s40691-021-00263-8
Dent, P. C. (2012). ‘Rare earth elements and permanent magnets’. Journal of Applied Physics 111(7), p.07A721. doi: https://doi.org/10.1063/1.3676616
Department for Science, Innovation and Technology (2023a). The UK Science and Technology Framework. [online] Available at: https://www.gov.uk/government/publications/uk-science-and-technology-framework/the-uk-science-and-technology-framework#identifying-critical-technologies [Accessed: 19 February 2025]
Department for Science, Innovation and Technology (2023b). National Vision for engineering biology. [online] Available at: https://www.gov.uk/government/publications/national-vision-for-engineering-biology [Accessed: 19 February 2025]
Department for Science, Innovation and Technology (2024a). Engineering biology public trust survey findings. [online] Available at: https://www.gov.uk/government/publications/engineering-biology-public-trust-survey-findings [Accessed: 25 March 2025].
Department for Science, Innovation and Technology (2024b). Engineering Biology Sandbox Fund. [online] Available at: https://www.gov.uk/government/publications/engineering-biology-sandbox-fund [Accessed: 7 March 2025]
Department for Science, Innovation and Technology (2024c). Press release – Science Secretary launches new Regulatory Innovation Office. [online] Available at: https://www.gov.uk/government/news/game-changing-tech-to-reach-the-public-faster-as-dedicated-new-unit-launched-to-curb-red-tape [Accessed: 7 March 2025]
Dixon, R. and Kahn, D. (2004). ‘Genetic regulation of biological nitrogen fixation’. Nat. Rev. Microbiol. 2, pp. 621-631. doi: https://dx.doi.org/10.1038/nrmicro954
Echavarri-Bravo V. et al (2022). ‘Selective bacterial separation of critical metals: towards a sustainable method for recycling lithium ion batteries’. Green Chemistry 24, pp. 8512-8522. doi: https://doi.org/10.1039/D2GC02450K
Echavarri-Bravo V. et al (2025). ‘Characterization and Reuse of Lithium-ion Battery Cathode Material Recovered Through a Bacterial Process’. Advanced Energy Materials (Early View). doi: https://doi.org/10.1002/aenm.202405901
Era Y. et al (2021). ‘Micellar catalysis of the Suzuki Miyaura reaction using biogenic Pd nanoparticles from Desulfovibrio alaskensis’. Green Chemistry 23, pp. 8886-8890. doi: https://doi.org/10.1039/D1GC02392F
Era Y. et al. (2022). ‘Palladium Nanoparticles from Desulfovibrio alaskensis G20 Catalyze Biocompatible Sonogashira and Biohydrogenation Cascades’. JACS Au, 2(11), pp. 2446-2452. doi: https://doi.org/10.1021/jacsau.2c00366
Erwin, S. I. (2010). How Much Does the Pentagon Pay for a Gallon of Gas? [online] National Defense. Available at: https://www.nationaldefensemagazine.org/articles/2010/4/1/2010april-how-much-does-the-pentagon-pay-for-a-gallon-of-gas [Accessed: 11 February 2025]
European Commission (2024). Reducing Emissions from Aviation. [online] Available at: https://climate.ec.europa.eu/eu-action/transport/reducing-emissions-aviation_en [Accessed: 11 February 2025]
European Parliament (2023). Parliament wants to make EU textiles and clothing industry greener. [online] Available at: https://www.europarl.europa.eu/news/en/press-room/20230524IPR91913/parliament-wants-to-make-eu-textiles-and-clothing-industry-greener [Accessed: 11 February 2025]
European Parliament (2024). The Impact of Textile Production and Waste on the Environment (infographics). [online] Available at: https://www.europarl.europa.eu/topics/en/article/20201208STO93327/the-impact-of-textile-production-and-waste-on-the-environment-infographics [Accessed: 11 February 2025]
FAO (2019). World fertilizer trends and outlook to 2022. [online] FAO. doi: https://doi.org/10.4060/ca6746en
Fashion for Good (2020) Financing the Transformation in the Fashion Industry. [online] Available at: https://reports.fashionforgood.com/wp-content/uploads/2020/10/FinancingTheTransformation_Report_FINAL_Digital-1.pdf [Accessed: 11 February 2025]
Faulkner, M. et al (2023). ‘Chemoautotrophic production of gaseous hydrocarbons, bioplastics and osmolytes by a novel Halomonas species’. Biotechnology for Biofuels and Bioproducts, 16(1). doi: https://doi.org/10.1186/s13068-023-02404-1
Food Standards Agency (2025). Press release - FSA launches pioneering regulatory programme for cell cultivated-products. [online] Available at: https://www.food.gov.uk/news-alerts/news/fsa-launches-pioneering-regulatory-programme-for-cell-cultivated-products [Accessed: 25 March 2025].
Geddes, B. A. et al (2015). ‘Use of plant colonizing bacteria as chassis for transfer of N2-fixation to cereals’. Curr. Opin. Biotechnol. 32, pp. 216-222. doi: https://doi.org/10.1016/j.copbio.2015.01.004
Geddes, B. A. et al (2019). ‘Engineering transkingdom signalling in plants to control gene expression in rhizosphere bacteria’. Nat. Commun. 10, 3430. doi: https://dx.doi.org/10.1038/s41467-019-10882-x
Giarratana, M.-C. et al (2011). ‘Proof of principle for transfusion of in vitro–generated red blood cells’. Blood, 118(19), pp. 5071–5079. doi: https://doi.org/10.1182/blood-2011-06-362038
Government Office for Science (2018). Futures, Foresight and Horizon Scanning Blog: 7 Questions Futures Technique. [online] Available at: https://foresightprojects.blog.gov.uk/2018/05/01/7-questions-futures-technique/ [Accessed: 3 March 2025]
Government Office for Science (2024). Futures toolkit for policymakers and analysts. [online] Available at: https://www.gov.uk/government/publications/futures-toolkit-for-policy-makers-and-analysts [Accessed: 3 March 2025]
Greene, S. et al (2020). ‘Well-to-tank carbon emissions from crude oil maritime transportation’. Transportation Research Part D: Transport and Environment, 88, p.102587. doi: https://doi.org/10.1016/j.trd.2020.102587
Haskett, T. L. et al (2022). ‘Engineered plant control of associative nitrogen fixation’. PNAS USA 119, e2117465119. doi: https://doi.org/10.1073/pnas.2117465119
Haskett, T. L. et al (2023). ‘Rhizopine biosensors for plant-dependent control of bacterial gene expression’. Environmental Microbiology 25, pp. 383-396. doi: https://doi.org/10.1111/1462-2920.16288
Haskett, T. L., Tkacz, A. and Poole, P. A. (2020). ‘Engineering rhizobacteria for sustainable agriculture’. The ISME Journal 15, pp. 949-964. doi: https://doi.org/10.1038/s41396-020-00835-4
Hawksworth, J. et al (2018). ‘Enhancement of red blood cell transfusion compatibility using CRISPR‐mediated erythroblast gene editing’. EMBO Molecular Medicine, 10(6), e8454. doi: https://doi.org/10.15252/emmm.201708454
Higgins, G. (2024). IATA reveals SAF production growth in 2024, urges acceleration to meet decarbonisation targets. [online] International Airport Review. Available at: https://www.internationalairportreview.com/news/232770/iata-reveals-saf-production-growth-in-2024-urges-acceleration-to-meet-decarbonisation-targets/ [Accessed 11 February 2025]
House of Lords, Science and Technology Committee (2025). ‘Don’t fail to scale - seizing the opportunity of engineering biology’. [online] Available at: https://committees.parliament.uk/committee/193/science-and-technology-committee/news/204667/uk-must-turbocharge-its-innovation-policy-to-harness-engineering-biology-say-peers/ [Accessed 11 February 2025]
IEA (2021). The Role of Critical Minerals in Clean Energy Transitions – Analysis. [online] Available at: https://www.iea.org/reports/the-role-of-critical-minerals-in-clean-energy-transitions [Accessed: 11 February 2025]
IPCC (2022). Frequently Asked Questions. Climate Change 2022: Mitigation of Climate Change. Available at: https://www.ipcc.ch/report/ar6/wg3/downloads/report/IPCC_AR6_WGIII_FAQs_Compiled.pdf [Accessed: 11 February 2025]
Jhu, M.-Y. and Oldroyd, G. E. D. (2023). ‘Dancing to a different tune, can we switch from chemical to biological nitrogen fixation for sustainable food security?’ PLOS Biology 21, e3001982. doi: https://doi.org/10.1371/journal.pbio.3001982
Kupzig, S. (2016). ‘Superior survival of ex vivo cultured human reticulocytes following transfusion into mice’. Haematologica, 102(3), pp.476–483. doi: https://doi.org/10.3324/haematol.2016.154443
Kyriakou, V. et al (2019). ‘An Electrochemical Haber-Bosch Process’. Joule 4(1), pp. 142 - 158. doi: https://doi.org/10.1016/j.joule.2019.10.006
Langlands, H.D., Shoemark, D.K. and Toye, A.M. (2024). ‘Modulation of Antioxidant Enzyme Expression of In Vitro Culture-Derived Reticulocytes’. Antioxidants, 13(9), pp.1070–1070. doi: https://doi.org/10.3390/antiox13091070
Leonard, S. V. L. et al (2024) ‘Microplastics in human blood: Polymer types, concentrations and characterisation using μFTIR’, Environment International, 188, pp.108751–108751. doi: https://doi.org/10.1016/j.envint.2024.108751
Lopez-Torrejon G. et al (2016). ‘Expression of a functional oxygen-labile nitrogenase component in the mitochondrial matrix of aerobically grown yeast’. Nat Commun 7, 11426. doi: https://dx.doi.org/10.1038/ncomms11426
McKinsey & Company (2020). The Bio Revolution: Innovations transforming economies, societies and our lives. [online] Available at: https://www.mckinsey.com/industries/life-sciences/our-insights/the-bio-revolution-innovations-transforming-economies-societies-and-our-lives [Accessed: 19 February 2025]
McKinsey & Company (2021). The UK biotech sector: The path to global leadership. [online] Available at: https://www.mckinsey.com/industries/life-sciences/our-insights/the-uk-biotech-sector-the-path-to-global-leadership#/ [Accessed: 19 February 2025]
Mehta, A. (2023). Despite climate pledges, fashion brands ‘way off track’ on cutting carbon from the catwalk. [online] Reuters. Available at: https://www.reuters.com/sustainability/climate-energy/despite-climate-pledges-fashion-brands-way-off-track-cutting-carbon-catwalk-2023-07-31/ [Accessed: 11 February 2025]
Meinders, M. et al (2020). ‘Expression and Retention of Thymidine Phosphorylase in Cultured Reticulocytes as a Novel Treatment for MNGIE’. Molecular Therapy - Methods & Clinical Development, 17, pp.822–830. doi: https://doi.org/10.1016/j.omtm.2020.03.029
Ministry of Defence (2022). RAF’s first synthetic fuel drone flight. [online] Available at: https://www.gov.uk/government/news/rafs-first-synthetic-fuel-drone-flight [Accessed: 11 February 2025]
Ministry of Defence (2024). Development of battle-winning hypersonic technology accelerated under new AUKUS deal. [online] Available at: https://www.gov.uk/government/news/development-of-battle-winning-hypersonic-technology-accelerated-under-new-aukus-deal [Accessed: 11 February 2025]
Moura, P.L. et al (2018). ‘Non-muscle myosin II drives vesicle loss during human reticulocyte maturation’. Haematologica, 103(12), pp.1997–2007. doi: https://doi.org/10.3324/haematol.2018.199083
Mus, F. et al (2016). ‘Symbiotic Nitrogen Fixation and the Challenges to Its Extension to Nonlegumes’. Applied and Environmental Microbiology 82, pp. 3698-3710. doi: https://doi.org/10.1128/AEM.01055-16
NHS (2022). First ever clinical trial of laboratory grown red blood cells being transfused into another person. [online] NHS Blood and Transplant. Available at: https://www.nhsbt.nhs.uk/news/first-ever-clinical-trial-of-laboratory-grown-red-blood-cells-being-transfused-into-another-person/ [Accessed: 11 February 2025]
Pakostova E. et al (2024). ‘A novel closed-loop biotechnology for recovery of cobalt from a lithium-ion battery active cathode material’. Microbiology 170, 001475. doi: https://doi.org/10.1099/mic.0.001475
Pantidos N. (2018). ‘Room-temperature bio-production, isolation and anti-microbial properties of stable elemental copper nanoparticles’. New Biotechnology, 40, pp. 275-281. doi: https://doi.org/10.1016/j.nbt.2017.10.002
Partington, R. (2022). Manufacturing’s Coming home: UK Fashion Boss Champions ‘reshoring’. [online] The Guardian. Available at: https://www.theguardian.com/business/2022/aug/06/uk-manufacturing-fashion-boss-champions-reshoring [Accessed: 11 February 2025]
Pellegrin, S., Severn, C.E. and Toye, A.M. (2021). ‘Towards manufactured red blood cells for the treatment of inherited anemia’. Haematologica, 106(9), pp.2304–2311. doi: https://doi.org/10.3324/haematol.2020.268847
Poole, P., Ramachandran, V. and Terpolilli, J. (2018). ‘Rhizobia: from saprophytes to endosymbionts’. Nature Reviews Microbiology, 16(5), pp.291–303. doi: https://doi.org/10.1038/nrmicro.2017.171
Ramanathan R. et al (2011). ‘Bacterial kinetics-controlled shape-directed biosynthesis of silver nanoplates using Morganella psychrotolerans’. Langmuir, 27 (2), pp. 714-719. doi: https://doi.org/10.1021/la1036162
Raykar, N.P. et al (2021). ‘Assessing the global burden of hemorrhage: The global blood supply, deficits, and potential solutions’. SAGE Open Medicine, 9, 205031212110549. doi: https://doi.org/10.1177/20503121211054995
Schäfer, A.W. et al (2018). ‘Technological, economic and environmental prospects of all-electric aircraft’. Nature Energy, 4(2), pp.160–166. doi: https://doi.org/10.1038/s41560-018-0294-x
Sciencewise (2024a). Public perceptions of engineering biology (food). [online] Available at: https://sciencewise.org.uk/2024/04/public-perceptions-of-engineering-biology-food/ [Accessed: 7 March 2025]
Sciencewise (2024b). Public perceptions of engineering biology (health). [online] Available at: https://sciencewise.org.uk/2024/01/public-perceptions-of-engineering-biology-health/ [Accessed: 7 March 2025]
Sivalingam, J. et al (2021). ‘A Scalable Suspension Platform for Generating High-Density Cultures of Universal Red Blood Cells from Human Induced Pluripotent Stem Cells’. Stem Cell Reports, 16(1), pp.182–197. doi: https://doi.org/10.1016/j.stemcr.2020.11.008
Smith, B.J. et al (2022). ‘Platinum Group Metal Catalysts: Supply Chain Deep Dive Assessment’. USDOE Office of Policy. doi: https://doi.org/10.2172/1871583
Steffen, W. et al (2015). ‘Planetary boundaries: Guiding human development on a changing planet’. Science [online] 347(6223). doi: https://dx.doi.org/10.1126/science.1259855
Tanner, L. et al (2024). Horizon Scan of the Artificial Blood Research and Funding Landscape. [online] NIHR Innovation Observatory, Newcastle University. Available at: https://www.io.nihr.ac.uk/wp-content/uploads/2024/09/Artificial-Blood-Report.pdf [Accessed: 11 February 2025]
Textile Exchange (2024). Synthetics. [online] Available at: https://textileexchange.org/synthetics [Accessed: 11 February 2025]
Thomsen, S. (2024). Synthetic biology is a $30 billion opportunity creating 50,000 jobs according to CSIRO and its VC arm. [online] Startup Daily. Available at: https://www.startupdaily.net/topic/other-tech/synthetic-biology-is-a-30-billion-opportunity-creating-50000-jobs-csiro-and-its-vc-arm-claim/ [Accessed: 11 February 2025]
Trakarnsanga, K. et al (2017). ‘An immortalized adult human erythroid line facilitates sustainable and scalable generation of functional red cells’. Nature Communications, 8(1), 14750. doi: https://doi.org/10.1038/ncomms14750
UK Government (2025). Department for Energy Security and Net Zero. [online] Available at: https://www.gov.uk/government/organisations/department-for-energy-security-and-net-zero [Accessed: 11 February 2025]
UK Parliament (2023). Genetic Technology (Precision Breeding) Act 2023. [online] Available at: https://bills.parliament.uk/bills/3167 [Accessed: 26 March 2025]
UK Research and Innovation (2023). Synthetic Biology for Growth. [online] Available at: https://www.ukri.org/what-we-do/browse-our-areas-of-investment-and-support/synthetic-biology-for-growth/#:~:text=The%20Synthetic%20Biology%20for%20Growth,DNA%20synthesis [Accessed: 19 February 2025]
UK Research and Innovation (2024). New £100m fund will unlock the potential of engineering biology. [online] Available at: https://www.ukri.org/news/new-100m-fund-will-unlock-the-potential-of-engineering-biology/ [Accessed: 7 March 2025].
UK SAF Clearing House (n.d.). Fuel test facilities. [online] Available at: https://www.safclearinghouse.uk/fuel-test-facilities [Accessed: 11 February 2025]
UNEP (2022). UN Alliance for Sustainable Fashion Addresses Damage of ‘Fast Fashion’. [online] Available at: https://www.unep.org/news-and-stories/press-release/un-alliance-sustainable-fashion-addresses-damage-fast-fashion [Accessed: 11 February 2025]
US Department of Energy (2023). Alternative Fuels Data Center: Sustainable Aviation Fuel. [online] Available at: https://afdc.energy.gov/fuels/sustainable-aviation-fuel [Accessed: 11 February 2025]
USDA (n.d.) What is the BioPreferred Program? [online] Available at: https://www.biopreferred.gov/BioPreferred/faces/pages/AboutBioPreferred.xhtml [Accessed: 11 February 2025]
Valckx, N. et al (2021). Metals Demand From Energy Transition May Top Current Global Supply. [online] IMF. Available at: https://www.imf.org/en/Blogs/Articles/2021/12/08/metals-demand-from-energy-transition-may-top-current-global-supply [Accessed: 11 February 2025]
Wright, T. (2023). Accelerating the UK’s high-speed missile ambitions. [online] International Institute for Strategic Studies. Available at https://www.iiss.org/online-analysis/military-balance/2023/08/accelerating-the-uks-high-speed-missile-ambitions/ [Accessed: 11 February 2025]